- 1Department of Agricultural Microbiology, Faculty of Agriculture, Zagazig University, Zagazig, Egypt
- 2Department of Biochemistry, Faculty of Agriculture, Zagazig University, Zagazig, Egypt
- 3Department of Internal Medicine and Infectious Diseases, Faculty of Veterinary Medicine, Cairo University, Giza, Egypt
- 4Department of Poultry Diseases, Faculty of Veterinary Medicine, Cairo University, Giza, Egypt
- 5Department of Agricultural Microbiology, Faculty of Agriculture, Beni-Suef University, Beni-Suef, Egypt
- 6Key Laboratory of Agro-Forestry Environmental Processes and Ecological Regulation of Hainan Province, Hainan University, Haikou, China
- 7Plant Production Department, Arid Lands Cultivation Research Institute, The City of Scientific Research and Technological Applications, Alexandria, Egypt
- 8Jiangsu Key Laboratory for Microbes and Genomics, School, of Life Sciences, Nanjing Normal University, Nanjing, China
- 9Department of Soils and Water, Faculty of Agriculture, Fayoum University, Fayoum, Egypt
- 10Department of Home Economic, Specific Education Faculty, Port Said University, Port Said, Egypt
- 11Department of Clinical Laboratory Sciences, College of Applied Medical Sciences, Jouf University, Sakaka, Saudi Arabia
- 12Medical Genetics Department, College of Medicine, Umm Al-Qura University, Makkah, Saudi Arabia
- 13Soil Science Department, Faculty of Agriculture, Zagazig University, Zagazig, Egypt
- 14Department of Biology, College of Science, United Arab Emirates University, Al-Ain, United Arab Emirates
- 15Khalifa Center for Genetic Engineering and Biotechnology, United Arab Emirates University, Al-Ain, United Arab Emirates
- 16Harry Butler Institute, Murdoch University, Murdoch, WA, Australia
Plant diseases and pests are risk factors that threaten global food security. Excessive chemical pesticide applications are commonly used to reduce the effects of plant diseases caused by bacterial and fungal pathogens. A major concern, as we strive toward more sustainable agriculture, is to increase crop yields for the increasing population. Microbial biological control agents (MBCAs) have proved their efficacy to be a green strategy to manage plant diseases, stimulate plant growth and performance, and increase yield. Besides their role in growth enhancement, plant growth-promoting rhizobacteria/fungi (PGPR/PGPF) could suppress plant diseases by producing inhibitory chemicals and inducing immune responses in plants against phytopathogens. As biofertilizers and biopesticides, PGPR and PGPF are considered as feasible, attractive economic approach for sustainable agriculture; thus, resulting in a “win-win” situation. Several PGPR and PGPF strains have been identified as effective BCAs under environmentally controlled conditions. In general, any MBCA must overcome certain challenges before it can be registered or widely utilized to control diseases/pests. Successful MBCAs offer a practical solution to improve greenhouse crop performance with reduced fertilizer inputs and chemical pesticide applications. This current review aims to fill the gap in the current knowledge of plant growth-promoting microorganisms (PGPM), provide attention about the scientific basis for policy development, and recommend further research related to the applications of PGPM used for commercial purposes.
Introduction
Plant pathogens and pests can have a large impact on agricultural productivity. Plant diseases reduce yields by 21–30% in several important crops worldwide (Savary et al., 2019). Meanwhile, certain plant pathogens have developed long-term resistance against chemical management (Lucas, 2011). Some economically important plant diseases have become more prevalent. Dependence on chemical pesticides has become one of the most pressing challenges to global environmental sustainability and public health (Fones et al., 2020). Because many of insecticides are difficult to break down into simpler components that are less dangerous, toxic residues remain in the soil; thus, posing health concerns (Gilden et al., 2010). Awareness of the environmental and health risks associated with synthetic chemical pesticides is highly recommended for sustainable crop management and less used chemicals (Donley, 2019).
Synthetic agrochemicals have been considered unsustainable, causing the quest for more environmentally friendly alternatives. The focus of modern agriculture research has turned to farm practices. Plant growth-promoting rhizobacteria (PGPR) are effective, environmentally safe, and non-toxic naturally occurring microorganisms than can serve as a promising alternative to chemical pesticides. Besides, environmental factors can affect agricultural productivity; thus, this may worsen the scenario in a variety of ways. We have many reasons to take serious actions toward plant disease control management to improve our health and reduce the effects of environmental stresses (Chaloner et al., 2021). Biological control provides one of the most economical and long-term effective strategies for managing plant diseases and reducing crop loss.
Recent advances in our understanding to plant growth-promoting microorganisms (PGPMs) warrant a proper scientific evaluation of the relationship between the properties of PGPMs and their impact on plant growth, yield, and resistance/tolerance to biotic and abiotic stresses. In addition, this review study builds on a growing body of literature concerning some potential implementations of PGPMs in sustainable agriculture. Here, the aim is to provide a state-of-knowledge review reporting the effects of PGPMs on plants and finding solutions to the challenges that face microbial biological control agents (MBCAs) when applied on a large scale compared with those of chemicals.
PGPR as promising biocontrol agents
Soil is a complex ecosystem containing various groups of microorganisms, including bacteria, fungi, protists, and animals (Müller et al., 2016). These microorganisms play key roles in plant development, nutrient regulation, and biocontrol activities. They settle in the rhizosphere and endo-rhizosphere of plants, where they use a variety of direct and indirect processes to support plant growth. Lyu et al. (2020) have stated that the phytomicrobiome (plant-associated microorganisms) can provide competitive, exploitative, or neutral alliances with plants; thus, affecting crop yield. Recently, scientists have looked deeply into employing beneficial PGPR to inhibit phytopathogens and promote plant growth (Qiao et al., 2017; Alwahshi et al., 2022). A key part of this might be attributed to the enhancement of target specificity between PGPR and the plant species (Lommen et al., 2019).
According to Zgadzaj et al. (2016), rhizosphere microbiome refers to bacterial, archaeal and fungal communities as well as their genetic material closely surrounding plant root systems. Microorganisms can indirectly impact crop health and phenotypic plasticity by influencing the growth of plants and defense responses due to their co-evolution with plants on a large scale (Goh et al., 2013). The rhizosphere is home to various microorganisms that provide steady PGPR supplies (Antoun and Kloepper, 2001). The phytomicrobiome includes the bacterial population that colonize the rhizosphere, on the root surface, and between the root cortex cells (Inui Kishi et al., 2017). Since plants can first colonize the terrestrial environments, PGPR have co-evolved with related plants; resulting in synergistic host plants’ relationships (Gouda et al., 2018). The effects, methods, and possibility for successfully applying PGPR to agricultural plant production in controlled situations have been the subject of numerous studies. This is critical for developing more widely used methods of biological control that consider field settings.
Safety and quality control are more crucial in vegetable cultivation since we use them less processed or unprocessed, and they have impact on health. PGPR are more achievable under greenhouse conditions. Because of the controlled environment, a significant number of prospective BCA has been discovered, and maybe ready for placement (Singh et al., 2017); thus, they have been confirmed to be successful in greenhouse investigations (Liu et al., 2018). Bacillus subtilis, Bacillus amyloliquefaciens and Pseudomonas stutzeri are among those shown to achieve success in root colonization as well as prevention of the pathogen Phytophthora capsici in cucumber (Islam et al., 2016). At the post-harvest stage, B. subtilis can protect tomato fruits from infection by Penicillium sp. and Rhizopus stolonifer (Punja et al., 2016).
Under greenhouse conditions, B. amyloliquefaciens isolates, diminish Fusarium oxysporum causing Fusarium wilt disease (Gowtham et al., 2016). In controlled situations, PGPR -as BCAs- are effective, indicating their role in greenhouse production systems and their efficacy in commercial horticulture. It is not necessary to distinguish the indirect PGPR pathways for pathogen infection avoidance and plant growth promotion under abiotic stresses. In addition, PGPR with biocontrol activities that also enhance plant growth would be more effective in practice. Plant tolerance to abiotic conditions and resistance to phytopathogens causing plant diseases can be improved by PGPR (Bhat et al., 2020; Leontidou et al., 2020). Some strains benefit plants’ coping with stress and flourishing in abiotic environments (Goswami and Deka, 2020).
While most researchers have reported PGPR under these controlled conditions, few of them have investigated their effectiveness as BCAs, especially when combined with an abiotic stress. This is a critical factor in field biocontrol, and when climate change affects the ecosystem. The long synergism between PGPR and plant may deliver various benefits to the host plant (Fan et al., 2020).
Biocontrol mechanisms using PGPR
PGPR can enhance the availability of certain nutrients [phosphate solubilization and nitrogen (N2) fixation], or synthesize the phytohormones [indole-3 acetic acid (IAA), ethylene (ET), jasmonic acid (JA), gibberellic acid (GA), and cytokinins (CKs); Mengiste et al., 2010; Vejan et al., 2016; Gouda et al., 2018; Sham et al., 2019].
PGPR colonizing a host plant can stimulate its growth through direct and indirect mechanisms (Figure 1). Direct mechanisms include the production of plant hormones, solubilization of phosphates, and increased uptake of iron. Indirect effects include antibiotics production, nutritional competition, parasitism, pathogen toxin inhibition, and induced resistance (Elnahal et al., 2022). The attitude of “PGPR” in creating phytohormones, molecules of signaling metabolites, and related substances describe how plants protect themselves from drought as an example of abiotic stress and salinity (Jochum et al., 2019). According to Abbas et al. (2019), PGPR may also alter the shape of the roots, resulting in increased root surface and improved root performance. In addition, PGPR can compete with other bacteria by colonizing rapidly and accumulating a greater supply of nutrients, preventing other organisms from growing (Salomon et al., 2017, Abd El-Mageed et al., 2020). PGPR have different strategies to colonize, of which each is tied to a particular host (Choudhary et al., 2011). In general, pathogen infections can be suppressed by using antibiotics and antifungal metabolites; thus considered a well-known direct biological control strategy (Raaijmakers et al., 2002). Bacteriocins, antibacterial proteins, and enzymes are examples of antimicrobial peptides (Compant et al., 2005). Antibiotics are small antimicrobial molecules produced by PGPR that can inhibit the process of metabolic or growth activities of microbial pathogens (Duffy et al., 2003). These antibiotics, which are mostly strain-specific, can target the ribosomal RNA (rRNA), alter the membrane structure, and damage the cell walls of bacterial pathogens (Abriouel et al., 2011; Maksimov et al., 2011; Nazari and Smith, 2020).
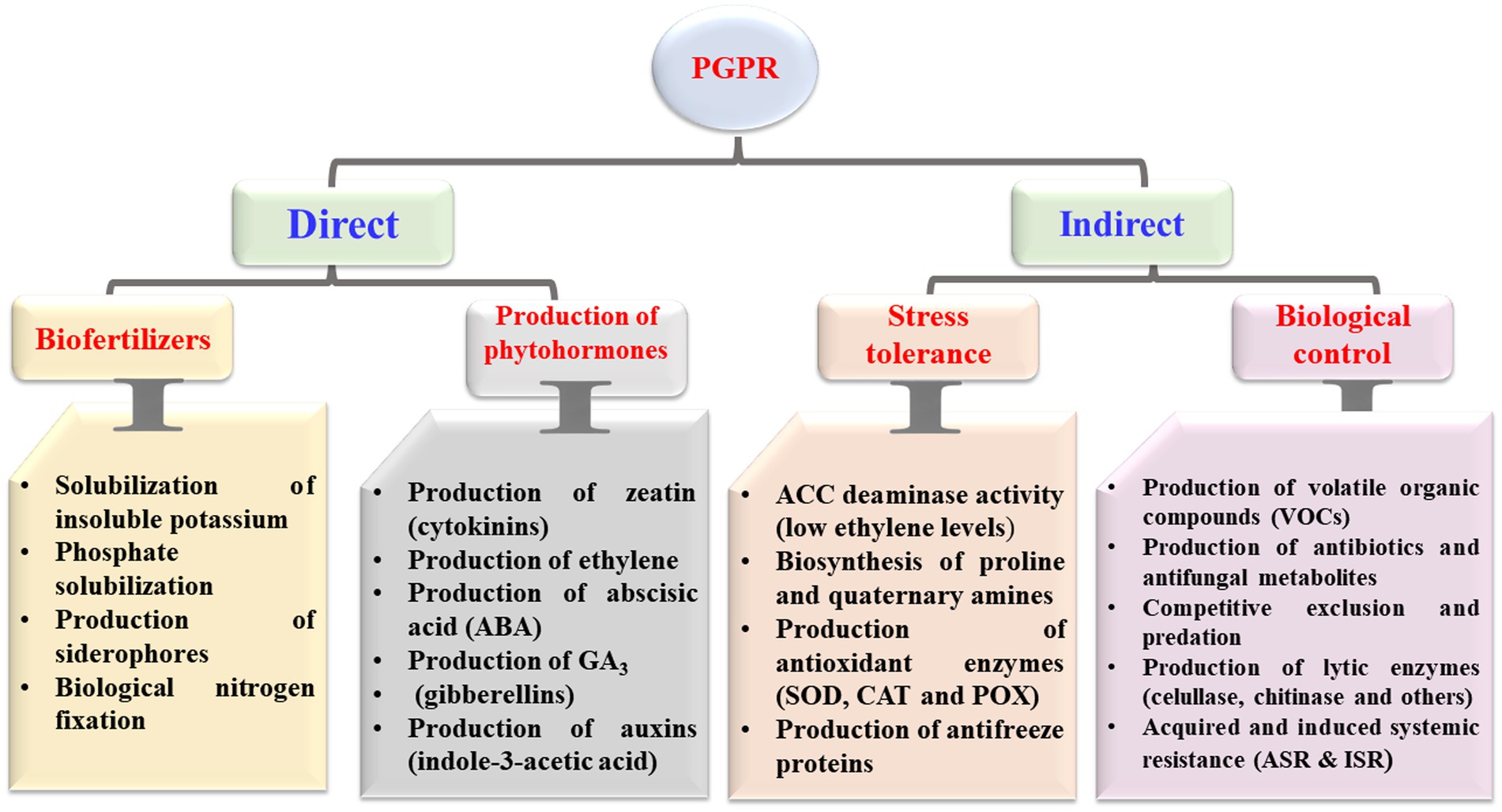
Figure 1. Direct and indirect mechanisms mediated by plant growth-promoting rhizobacteria (PGPR) with beneficial effects on host plants. ACC, 1-aminocyclopropane-1-carboxylic acid; SOD, superoxide dismutase; CAT, catalase; and POX, peroxidase.
Many bacteria produce bacteriocins, where some have a greater variety of inhibitory activities than others (Abriouel et al., 2011). Siderophores are specialized chelating agents of ferric iron that inhibit phytopathogens from gaining access to iron; thus, maintaining plant health particularly in iron-deficient environments (Shen et al., 2013). PGPR can manage various plant diseases by depriving pathogens of iron, thereby reducing disease development and generating extracellular siderophores (Radzki et al., 2013). Bacteriocins, siderophores, and antibiotics have thus been identified as the three supreme operative approaches for potential biocontrol prior to in vivo applications (Kloepper et al., 1980). Several studies have investigated PGPR as a potential plant disease management tool to synthesize plant-beneficial metabolites such as siderophores (Subramanian and Smith, 2015).
PGPR can indirectly increase crop stress tolerance. Signal chemicals, such as phytohormones and specialized signal molecules, enable plant-to-microbe and microbe-to-plant communication (Lyu et al., 2020). The control and regulation of activities in the holobiont include the host plant and the “specific” phytomicrobiome (the plant-phytomicrobiome interaction). Two microbe-to-plant signals, lipo-chitooligosaccharides (LCOs) and thuricin17 (TH17), enhance stress tolerance in different plant species (Lyu et al., 2020). Resistance-inducing and antagonizing PGPR might be useful as new inoculants with combinations of different mechanisms of action, leading to a more efficient use for biocontrol strategies and plant growth promotion (Glick, 1995).
PGPR can also produce volatile organic compounds (VOCs) that play a significant role in plant growth and induced systemic resistance (ISR) to pathogens (Raza et al., 2016). Beneduzi et al. (2012) found that PGPR can trigger ISR as a strategy to improve disease resistance of plants. Roots colonization by arbuscular mycorrhizal fungi (AMF) and certain strains of non-pathogenic bacteria can improve plant resistance to biotic stresses (Mauch-Mani et al., 2017; Pérez-de-Luque et al., 2017). ISR triggered by PGPR and plant growth-promoting fungi (PGPF) can be found in a wide range of plant taxa (Bhattacharyya and Jha, 2012). Systemic acquired resistance (SAR); however, can be activated by a pathogen infection (Gao et al., 2015). Salicylic acid (SA) signaling is associated with the production of pathogenesis-related (PR) genes. Unlike SAR, ISR functions independently of SA, but requires responses to ET and JA. This can be achieved by the induction of defense-related gene expression; although is not always associated with induced PR proteins (Mathys et al., 2012).
Previous studies have identified ISR to stimulate PGPR via the SA-dependent pathway rather than the JA/ET-dependent pathway (Takishita et al., 2018). Other plant hormones, such as auxins, GA, CKs and brassinosteroids, may also contribute to plant immunity (Nakashita et al., 2003; Kazan and Manners, 2009; Giron et al., 2013; Rady et al., 2021). Hormonal crosstalk is thought to allow the cultivation and exert their immunological growth and defense reactions (Pieterse et al., 2014; AbuQamar et al., 2017; Sham et al., 2017).
PGPR are involved in diverse mechanisms to enhance plant growth and/or act as BCAs. Crop production promotion and disease management could be investigated together to ensure sustainability and cost-effectiveness of agricultural systems. Thus, effective PGPR strains can promote stress tolerance and nutrient absorption, plant development, and battling fungal/bacterial diseases. Thus, this appears to be a win-win situation to the PGPR strain and the host plant.
Challenges of employing PGPR as BCAs
PGPR-based biocontrol provides effective and long-lasting disease management. Europe and the United States are the most promising marketplaces for biocontrol products, followed by South America (Barratt et al., 2018). Although many PGPR have been tested in vitro and commercially proven as BCAs, new biocontrol products have been released from research activities carried out in the United States and Europe (Glick, 2012; O’Brien, 2017; Rosier et al., 2018). In general, the market of BCAs and their products is growing; yet, it is not well-adopted compared with chemical pesticides as the most common crop management method (Mishra et al., 2015).
Before being publically accepted/registered as a commercial BCA, there are certain requirements/needs that have to be taken into consideration (Bashan et al., 2014). As such, researchers should improve the efficacy of BCAs to manage certain disease(s). This can be achieved by having a BCA that has as many beneficial characteristics and mechanisms of action as possible. Such characteristics may include, but not limited to, the ability of the BCA to grow fast in vitro, produce a wide range of bioactive metabolites, possess high rhizosphere competence abilities, enhance plant growth performance, be environmentally safe, have the compatibility with other rhizobacteria/fungi, and be tolerant to abiotic stresses (Lyu et al., 2020). Successful colonization of root tissues and/or the rhizosphere is a critical component for any PGPR strain to be an effective BCA; thus, to perform well against plant pathogens. On the other hand, performance of the inoculated PGPR may vary, depending on the survival rate in the soil, crop compatibility, interaction with other local microbial species and the environmental factors (Vejan et al., 2016). Survival and colonization are major components when identifying effective BCA isolates. In vitro antagonism experiments are often used to investigate the effect of bacterial isolates on certain diseases, prior to greenhouse and/or field trials (Bashan et al., 2014).
Performance of PGPR is generally assessed according to the geographical areas, soil types, host crop species, and under various environmental conditions (Choudhary et al., 2011). BCA growth is often easier to monitor under controlled conditions, i.e., greenhouses. The preference of this stage by most researchers could be attributed to the stability of environmental conditions. Greenhouse experiments evaluating the performance of BCAs under controlled conditions can provide strong theoretical and practical support for the application of PGPR in the field. Thus, this ensures the feasibility and efficacy of PGPR for commercial horticulture production, disease management and climate change conditions such as those found under field conditions.
PGPR stability is also influenced by the method, formulations, transportation, and storage conditions. To achieve high levels of the BCA survival (McIntyre and Press, 1991), one should improve the formulation technology (Lobo et al., 2019), increase the shelf-life of the BCA product (Carrasco-Espinosa et al., 2015), optimize the production of targeted microbial types (Zhang et al., 2019) and achieve low-cost production at large scales (Kang et al., 2017). Many scientists have attempted to extend the shelf-life of PGPR by decreasing the storage temperature and/or modifying the combinations of additives (Lee et al., 2016; Berger et al., 2018). Extensive research on the risks and benefits of BCAs is also required, because agricultural disease management approaches rely on this balance.
Due to the diverse modes of action, identification, characterization, the registration of promising PGPR strains take time and require academic-industry collaborations. Using natural sources (e.g., BCAs) to control pathogens also poses a set of legal and ethical issues that may threaten the local biodiversity (Hajek et al., 2016). In that regard, new species/populations of BCAs have been restricted from entering specific countries. For commercial uses, the application of PGPR in protected environments such as greenhouses is much easier, due to a more isolated and controlled environment delivery and potentially less negative ecological consequences. Another challenge that is linked to the widespread implementation of PGPR-based biocontrol is the regulatory problems. Currently, each country has its regulatory system that greatly vary among them (Bashan et al., 2014).
High development costs for new commercial BCAs, for example, have been identified as a barrier to the BCA industry’s expansion in Australia (Begum et al., 2017). The high regulatory expenses of importing new BCAs into Australia is one of the most serious challenges. BCA registration requies tight coordination among governmental institutes, universities, and industrial sectors to facilitate the assessment and commercialization of new BCAs and their products. The shortage of programs for financial and ecological benefits can also be added as a challenging problem (Heimpel et al., 2013). For global marketing and local practical applications, commercialization should follow international legislation. The International Biological Control Organization (IOBC) have gathered academicians, researchers and practitioners from different sectors/fields to identify the barriers and provide recommendations to overcome these limitation (Barratt et al., 2018).
When compared to chemical pesticides, which are more reliable and predictable, farmers could notice little or no economic gain. Such programs, including local seminars, training workshops and free conferences may increase awareness about the application of BCA in specific farming areas. Finally, PGPR-based biocontrol can hold a lot of promises to reduce agrochemicals use in agriculture. The widespread use of PGPR as BCAs requires massive effort from regulatory bodies and crop growers to convince the public and earn their trust in the capacity of the new BCA products to manage diseases and increase crop yields. High-value crop production in greenhouses could be an ideal place to test the efficacy of PGPR as BCAs in response to different abiotic stresses. Based on recent successful greenhouse trials, BCAs can be used in the field for managing disease and associated agricultural plant growth enhancement (Alwahshi et al., 2022).
Rhizobacteria as BCAs
In the past few decades, rhizobacteria have gained attention when applied to grains, seeds, roots, and/or soils to help the plant grow and develop. Rhizobacteria are important for N2 fixation, promotion of plant growth, and biological control of plant pathogenic microorganisms. Recently, various microbial species are presently used in bacterization, containing Azospirillum, Azotobacter, Bacillus, Rhizobium, Serratia, Stenotrophomonas, Streptomyces Acinetobacter, Agrobacterium, Alcaligenes, Arthrobacter, Bradyrhizobium, Frankia, Pantoea, Pseudomonas, and Thiobacillus (Whipps, 2001). Many plant diseases associated with nematodial, bacterial and fungal infections have been reported to be managed by PGPR. The use of BCAs has been controversial in suppressing nematode populations because other soil microorganisms and the host plant can be adversely affected. To manage diseases associated with plant-parasitic nematodes a combination of biological management, nematicides, organic soil amendments, and crop rotation have been used (Timper, 2011). In vitro culture filtrates of a strain of Pseudomonas sp. can suppress juvenile mortality of Meloidogyne javanica; thus, considerably reduce root gall and nematode population, and enhance plant development and yield (Nasima et al., 2002). Furthermore, inoculations with Bacillus spp. affect nematode behavior and feeding (Viaene et al., 2006). Pseudomonas striata, Pseudomonas fluorescens, and B. subtilis strains also overturn the population of nematodes (Table 1; Khan et al., 2012). Root-knot nematode (RKN) infestations have been successfully managed via biological management using Bacillus isolates (Lee and Kim, 2016). Few studies have reported the endophytic P. fluorescens and Bacillus spp. to promote systemic resistance in crops against nematodes owing to the increased activities of phenylalanine ammonia-lyase, polyphenol oxidase, and peroxidase, as defense-related enzymes for producing antagonistic chemicals and altering explicit root exudates such as amino acids and polysaccharides (Abbasi et al., 2014). P. fluorescens isolates increased defense enzymes in tomatoes resistant to RKN (Kavitha et al., 2013). In comparison with the control, the application of P. fluorescens and Paecilomyces lilacinus resulted in low nematode community in roots, tubers and soils (Mohan et al., 2017).
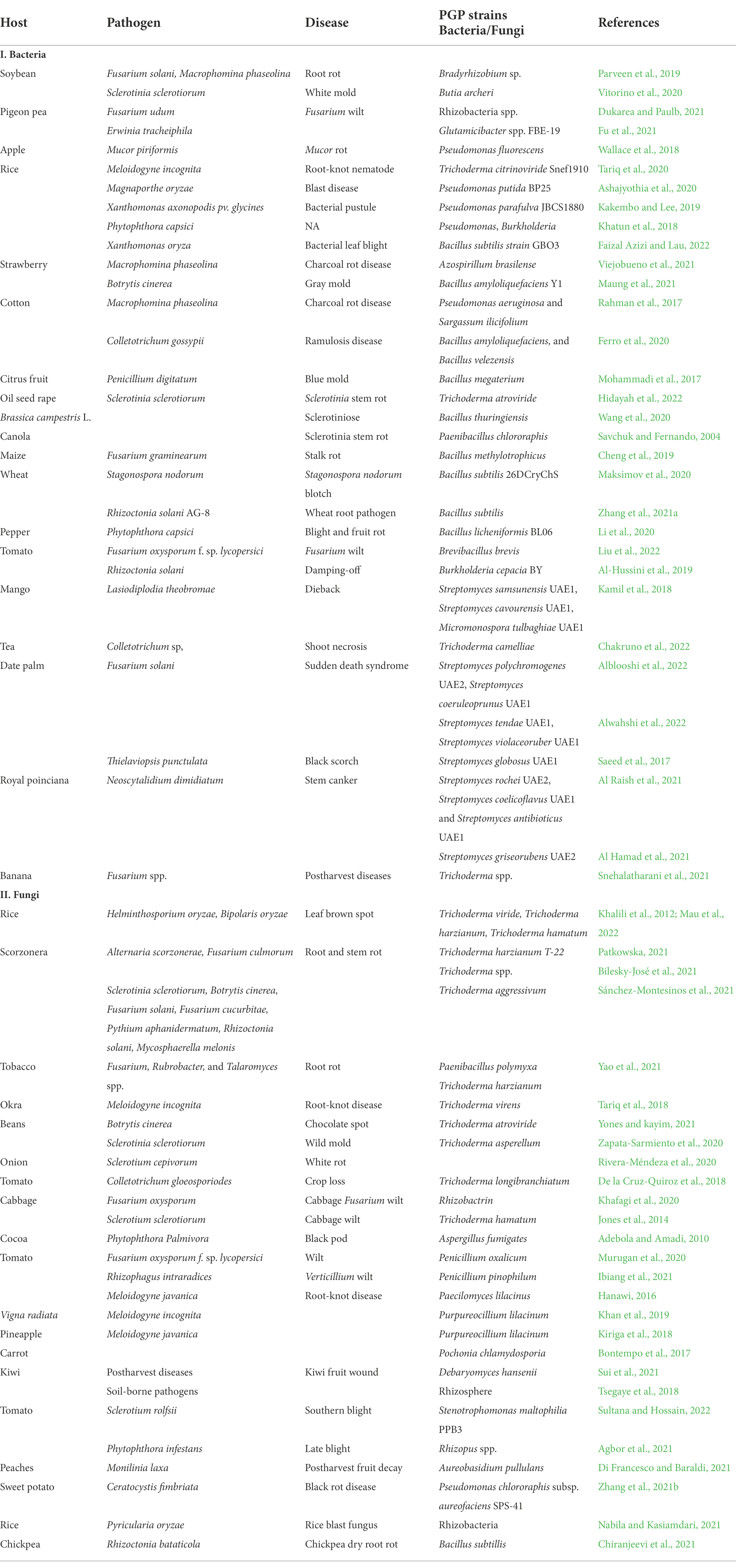
Table 1. Bacterial and fungal plant growth-promoting strains used as biocontrol agents against plant pathogenic microorganisms.
According to Jha et al. (2015), losses resulting from post-fresh fruit and vegetable harvest in India ranged between 4.6% and 15.9%. Although fungicides can inhibit the growth of phytopathogens, their use causes problems to the environment as well as the human and animal health (Nunes, 2012). The most environmentally acceptable practice to control post-harvest fungal diseases is by using BCAs. In general, BCAs can protect plants from fungal diseases, and are currently a viable option to manage post-harvest diseases associated with plant pathogens (Ghazanfar et al., 2016). In agriculture, BCAs can offer a number of advantages, including the reduction in the causing agents, farming preservation, minimum labor, soil, water plant contamination, and waste management difficulties (Torres et al., 2016). Fungal species, such as Alternaria, Aspergillus Penicillium, and Fusarium producing mycotoxins, are harmful to green vegetables and cause post-harvest diseases. Mycotoxins, such as fumonisin, ochratoxins, aflatoxins and other toxins, are released in vegetables and fruits infected with the fungal pathogens, Fusarium, Alternaria, and Aspergillus (Sanzani et al., 2016). The use of biopriming and pelletizing techniques of Serratia plymuthica HRO-C48 alongside Verticillium dahliae in canola plants revealed a significant biocontrol (Muller and Berg, 2008); thus, providing evidence of the ability of BCAs to manage diseases comparable to chemical fungicides.
Bacillus spp. produce a variety of compounds involved in the biocontrol of phytopathogens on various plants, including potato, rice, tomato, wheat, groundnut, brinjal, chickpea, and cucumber (Peng et al., 2014). Bacillus sp. BS061 isolate can mitigate the effect of Botrytis cinerea to reduce the occurrence of gray mold and powdery mildew diseases in strawberry and cucumber (Kim et al., 2013). Park et al. (2013) found that Pectobacterium carotovorum SCC1 can manage soft rot disease in tobacco plants when conjugated with B. subtilis strain B4 and BTH fungicides. The root-knot and root rot pathogens are often suppressed when Pseudomonas spp. are used as BCAs (Habiba et al., 2016). Farhat et al. (2017) revealed that PGPR isolates had antifungal activities in mungbean plants against Rhizoctonia solani, Macrophomina phaseolina, F. solani, and F. oxysporum. These isolates can also be used to prevent fungal infections that cause root rot disease. The application of the bacterial BCA, Pseudomonas aeruginosa, can manage anthracnose in the chili pepper against the causal pathogen Colletotrichum capsici (Jisha et al., 2018). P. aeruginosa can also induce systemic resistance of chili pepper to anthracnose.
Synthetic chemical pesticides are mainly used for post-harvest disease management. Thus, this may lead to plant pathogen resistance, soil deterioration, and toxicological hazards for the humans and the environment. Nowadays, a general trend, as a result, has been shifted toward finding an alternative to the use of agrochemicals in plant disease management. Compared to synthetic chemical fungicides, the use of microbial antagonists or BCAs has become a “hot” topic due to the numerous advantages as non-hazardous, green, economical and feasible applications to control post-harvest pathogen infections (Bonaterra et al., 2012).
Fungi as BCAs
Fungal BCAs are able to antagonize plant pathogens and protect their host plants. For example, several strains of Trichoderma have been developed as BCAs against the fungal pathogens Penicillium, Fusarium, Aspergillus, Alternaria, Pythium, Rhizoctonia, Phytophthora, Pyricularia, Botrytis, and Gaeumannomyces (Pal and Gardener, 2006; Adebola and Amadi, 2010; Agarwal et al., 2011; Alam et al., 2011; Nally et al., 2012). As a BCA, Trichoderma can suppress various air- and soil-borne plant pathogens; thus, can be conceivably used as biopesticides in greenhouse and/or field trials. According to Silva et al. (2017), certain strains of nematophagous fungi can manage the populations of Meloidogyne enterolobii in an integrated pest management (IPM) approach. AMF could also protect crops against soil-borne pathogens, including RKN, albeit the unclear mechanisms of antagonism (Vos et al., 2012).
The use of nematophagous and endoparasitic fungi has been deployed as antagonists to suppress RKN (Pendse et al., 2013). The talc-based formulation of the fungal BCA, Paecilomyces lilacinus, was found to be more active in reducing the population of Meloidogyne incognita in soils cultivated with tomato plants (Priya and Kumar, 2006). The efficiency of P. lilacinus in controlling nematodes was observed in several horticultural crops, including tomato, okra, and capsicum (Rao, 2007). The most widely used BCA for plant-parasitic nematodes is the fungus P. lilacinus, which has shown an appropriate replacement to synthetic chemical control in pre- and post-planting applications (Atkins et al., 2005). P. lilacinus infects eggs, juveniles and females of M. javanica by direct hyphal penetration (Esfahani and Pour, 2006). P. lilacinus can boost tomato yield while reducing the population of M. incognita in the soil and on the roots (Kalele et al., 2010). RKN management can also be achieved by using P. lilacinus and Bacillus firmus either individually or in combination. However, the mixture of P. lilacinus and B. firmus applied in soils 2 weeks prior to tomato transplantation showed the best practice to control Meloidogyne spp. (Anastasiadis et al., 2008).
Coating the seed with Trichoderma viride and P. lilacinus effectively reduced the nematode population in the soil. Species of Aspergillus and Paecilomyces were found to be antagonistic to M. incognita when compared to the single bio-agent treatment; thus, resulting in enhanced plant growth (Table 1; Bontempo et al., 2017). Kerry and Hidalgo-Diaz (2004) developed a management technique using the nematophagous fungus Pochonia chlamydosporia to manage RKN for the purpose of organic vegetable production. Okra seeds treated with Trichoderma harzianum, T. viride, P. lilacinus, P. chlamydosporia, and P. fluorescens at 20 g kg−1 seed signifcantly reduced the nematode population in the soil and promoted plant growth development (Kumar et al., 2012). Sharf et al. (2014) have reported that P. chlamydosporia exhibited nematicidal effects against M. incognita on infected common bean under greenhouse condition. Trichoderma spp. synthesizing chitinases, lytic enzymes, proteases, and glucanases were found to manage vegetable crop diseases (Punja and Utkhede, 2003). T. harzianum, T. viride, and T. hamatum have nematocidal properties when they colonize the roots of host plant and enhance their growth performance (Girlanda et al., 2001; Siddiqui and Shaukat, 2004; Zhang and Zhang, 2009). Because crop yield is mainly influenced by climatic conditions, agronomic factors, pests, and nutrient availability in the soil (Harman et al., 2004; Elrys et al., 2019a, 2020a), researchers must consider these factors in the selection of fungal BCAs.
Likewise, Trichoderma spp. can prevent nematode penetration and development in plants through the regulation of metabolites (Bokhari, 2009). Usman and Siddiqui (2012) have shown that the M. incognita and other RKN are more affected by the culture filtrate of Trichoderma. Species of Trichoderma can produce viridin, a nematicidal chemical (Watanabe et al., 2004). Gliotoxin and acetic acid, have also been reported as nematicidal substances in the culture filtrates of T. virens and T. longibrachiatum, respectively (Anitha and Murugesan, 2005). In response to M. incognita, T. polysporum has the ability to synthesize cyclosporine, the peptide that has a nematicidal action (Li et al., 2007). The efficacy of P. lilacinus, as bioagents or bioproducts in mixtures, significantly decreased the number of M. incognita on eggplant (Hanawi, 2016). It has been shown that different sources of N and carbon affect the growth and antagonism of Trichothecium roseum and T. viride (Arya, 2011). Although fructose and lysine were mostly effective against T. viride, rhamnose and glycine were more effective against T. roseum. There is an adverse effect of fungal culture filtrates on the egg hatching and juvenile mortality of RKN. Plants treated with the fungal BCA, Lecanicillium muscarium, decreased the number of galls in plants, eggs, juveniles (J2) and the reproduction factor (Rf) of Meloidogyne hapla compared to control plants (Hussain et al., 2017). In addition, plant growth was greatly improved when treated with L. muscarium. Trichoderma and P. lilacinum isolates dramatically reduced nematode egg number and mass, minimized root gal injury, and improved plant root mass development when compared to control plants without the fungal BCAs (Kiriga et al., 2018). Overall, more than 30 genera and 80 species of fungi can parasite RKN (Gaziea-Soliman et al., 2017). Prince et al. (2011) have demonstrated that the fungus, Colletotrichum falcatum, has antagonistic potential against the fungal pathogens Penicillium citrinum, Botrytis cinerea, and Trichodermum glaucum. Moreover, other fungi, such as Ampelomyces speciosus and Acremonium alternatum, have the ability to degrade the mycelia of fungal pathogens, indicating that not only rhizobacteria, but also fungi can serve as BCAs (Kiss, 2003).
Mechanisms used by MBCAs
Understanding the appropriate conditions for implementing proper programs against plant pathogens requires collaborations between different research groups focusing on the mechanisms associated with MBCAs to manage diseases on plants. In the last two decades, extensive research has focused on the antifungal effect, rhizosphere colonization, and crop benefits linked to MBCA (Compant et al., 2010; Al Hamad et al., 2021; Al Raish et al., 2021; Alwahshi et al., 2022). Thus, the products of MBCA on plant fungal pathogens and their impacts on plants are illustrated in Figure 2. The primary strategy of MBCA are summarized as antibiosis, competition for micronutrients such as iron, mycoparasitism, production of hydrolytic enzymes, and induction of ISR in host plants (Figure 3). In addition, the production of metabolites that are inhibitory to plant pathogenic rhizosphere microorganisms is considered one of the major biocontrol activities in many MBCA (Haas and Keel, 2003).
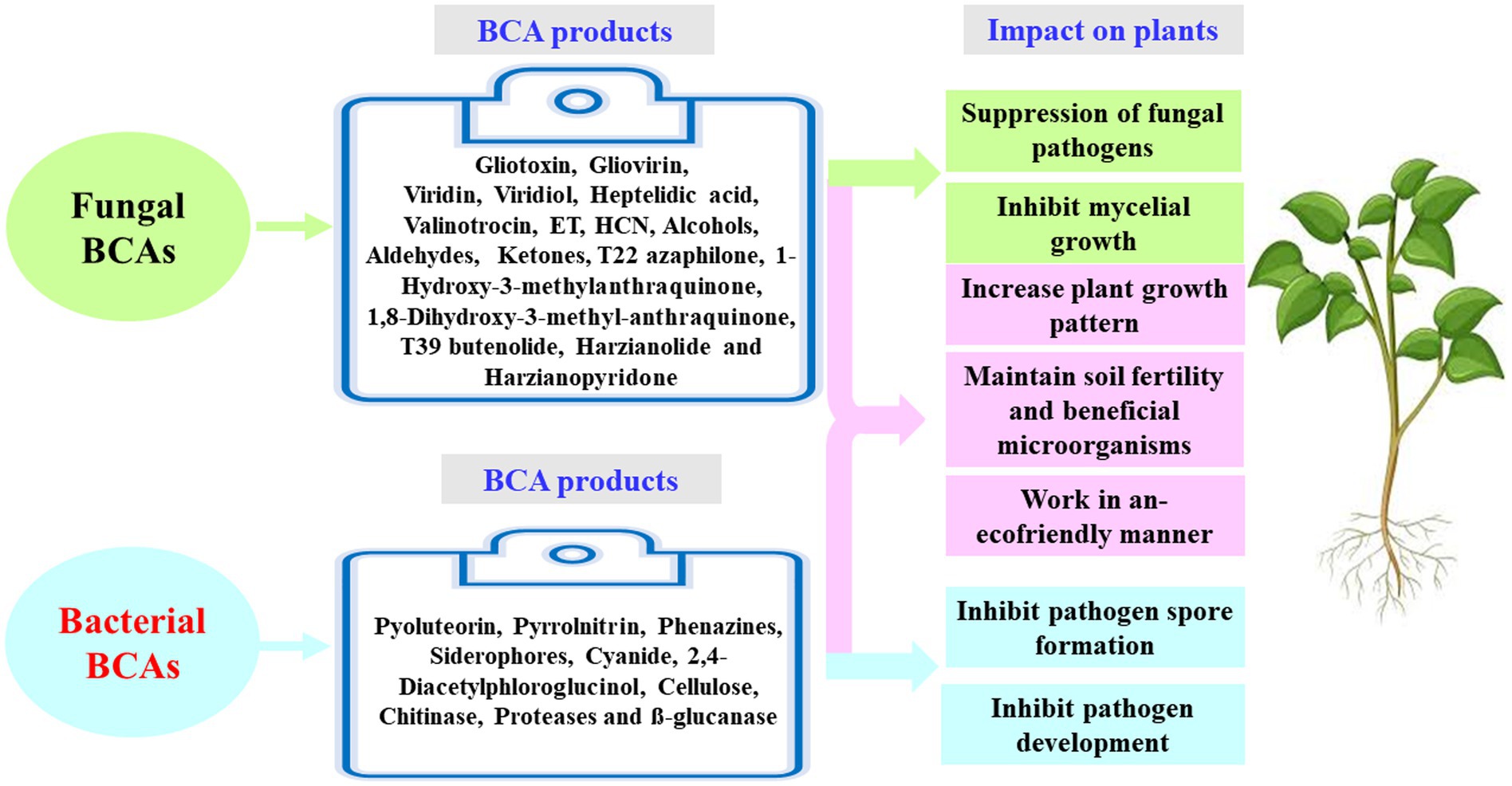
Figure 2. Fungal and bacterial biocontrol agents (BCAs) and their impact on plant. ET, ethylene; HCN, hydrogen cyanide.
In several microorganisms, antibiosis, also known as secondary metabolites, results in the production of various toxic chemicals to pathogenic microorganisms; thus, they are suitable for the plant growth and development. An antibiotic-producing microorganism must manufacture the antibiotic in the correct microniche on the root surface to effectively control plant diseases (Lugtenberg and Kamilova, 2009). Actinobacteria (8,700 distinct antibiotics), bacteria (2,900), and fungi (4,900) can produce massive amounts of antibiotics (Bérdy, 2005). Mutagenesis has been reported to be successful in determining the role of antibiotics generated by bacterial BCA isolates to control pathogens associated with plant infections (Liu et al., 2007).
Ongena and Jacques (2008) have investigated the lipopeptides (surfactin, iturin, and fengycin) in Bacillus spp. It has also been reported that pyrrolnitrin, 2,4-diacetylphloroglucinol (DAPG), and phenazine can be potential antibiotic metabolites in Pseudomonas (Raaijmakers and Mazzola, 2012). Pseudomonas spp. have the ability to generate pyoluteorin, siderophores, and cyanide, among other antimicrobial chemicals (Compant et al., 2010). In addition, the enzymatic activity of cellulase, proteases, β-glucanase, and chitinase can lyse fungal cells (Hernandez-Leon et al., 2015). Antibiotic metabolites produced by Pseudomonas spp. are regulated by complex regulatory networks and high number of transcription factors (Berry et al., 2014). Significant classes of antifungal antibiotics are lipopeptides or peptides that are produced by the ribosomes or non-ribosomes of Bacillus spp. (Figure 2). Arseneault and Filion (2017) have discussed that antibiotics can be generated by BCA strains in soil.
Bacillus spp. have the ability to produce various biologically active chemicals that hinder the development of several crop diseases (Zhao et al., 2013). An investigation by Chowdhury et al. (2015) revealed that the quantity of antibacterial or antifungal chemicals produced by Bacillus spp. in the rhizosphere is somewhat little, causing doubts on the role of rapid management of plant diseases. Several isolates of P. fluorescens were found to generate cyclic lipopeptides (CLPs), such as viscosinamide, amphisin and tensin, that were effective against fungal pathogens, R. solani and Pythium ultimum (Nielsen et al., 2002).
Biological control is an application of beneficial organisms, genes, and their products in the form of metabolites (Glare et al., 2012). Several in vitro metabolites of microorganisms were utilized to control pathogenic infections (Köhl et al., 2019). As a result, these secondary metabolites can be utilized as products of a BCA; and thus, they are effective to ameliorate the negative impact of other pathogenic microorganisms while also being environmentally friendly. Antimicrobial activities of some fungal BCAs may also exhibit antagonistic effects against fungi. For example, Trichoderma spp. are commonly found in soil and provide a variety of volatile and nonvolatile compounds. Volatile compounds, such as cyanide, hydrogen, ET, aldehydes, ketones and alcohols; and nonvolatile substances, such as peptides, can inhibit the mycelial growth in some pathogenic fungi. Many antifungal compounds, such as gliovirin, gliotoxin, viridiol, heptelidic acid, valinotrocin, and viridin can be produced by Gliocladium virens, which acts as a MBCA. Singh et al. (2005) demonstrated that gliotoxin can effectively reduce the fungal pathogens, Pythium aphanidermatum, M. phaseolina, Pythium debaryanum, R. solani, Sclerotium rolfsii, and Rhizoctonia bataticola. Vinale et al. (2009) have stated that the production of 1-hydroxy-3-methylanthraquinone, 1,8-dihydroxy-3-methyl-anthraquinone, T22 azaphilone, harzianolide, T39butenolide, and harzianopyridone by T. harzianum strains T22 and T39 has the ability to control the plant fungal pathogens Leptosphaeria maculans, Phytophthora cinnamomi, R. solani, Botrytis cinerea, and P. ultimum. Several secondary metabolites have been isolated and recognized by different methods such as high-performance liquid chromatography (HPLC) and gas chromatography–mass spectrometry (GC–MS). Shanthiyaa et al. (2013) have investigated three isolates, Cg-5, Cg-6, and Cg-7, that produce the secondary metabolite, chaetoglobosin A, in the culture filtrate detected by the UV spectrum at 250 nm. The antimicrobial compounds released by fungi may also control phytopathogens during post-harvest infections. The post-harvest infection causes excessive damages in vegetables and fruit (Figures 4, 5).
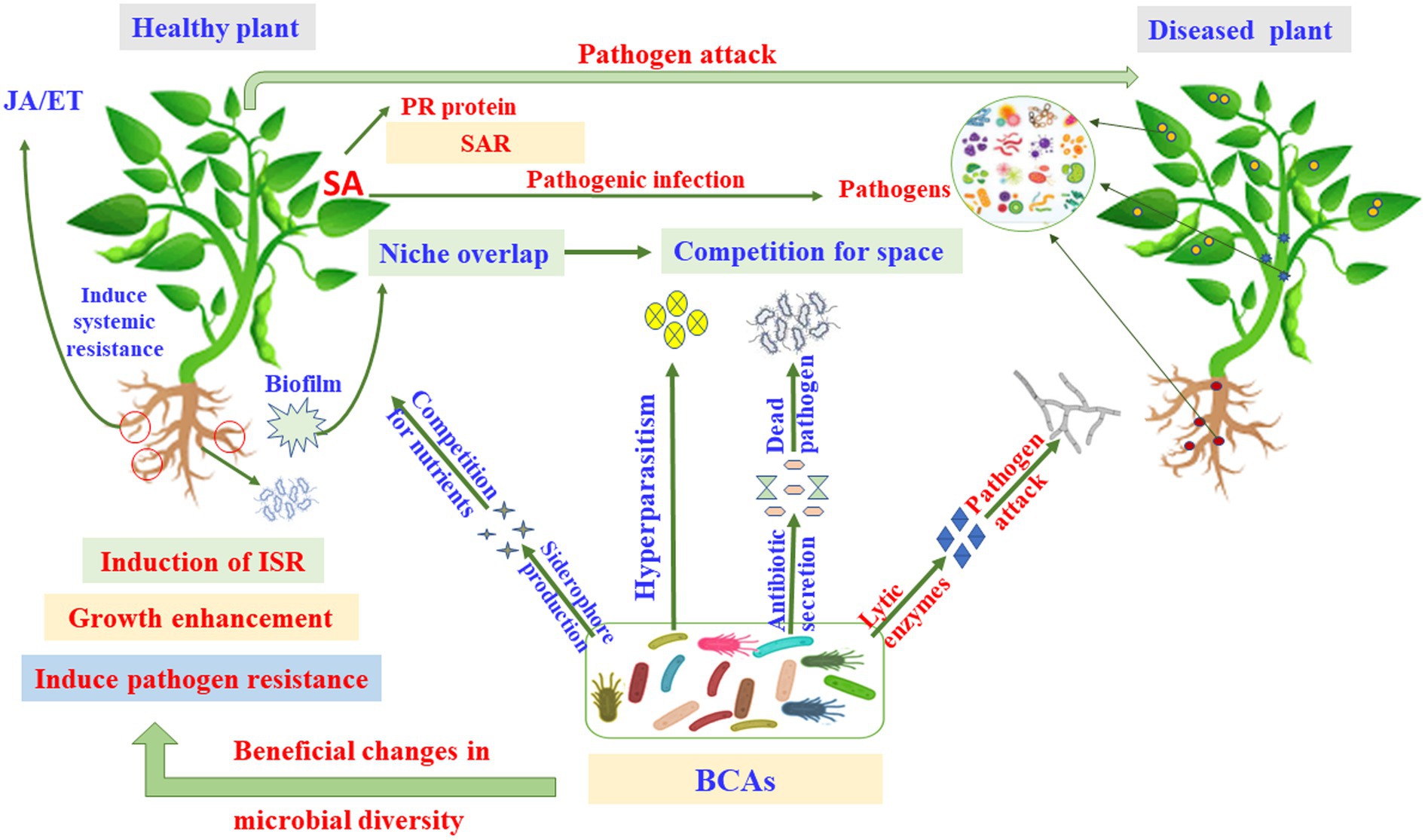
Figure 4. Pathways and modes of action of microbial biocontrol agents (MBCAs). ET, ethylene; ISR, induced systemic resistance; JA, jasmonic acid; PR, pathogenesis-related; SA, salicylic acid; SAR, systemic acquired resistance.
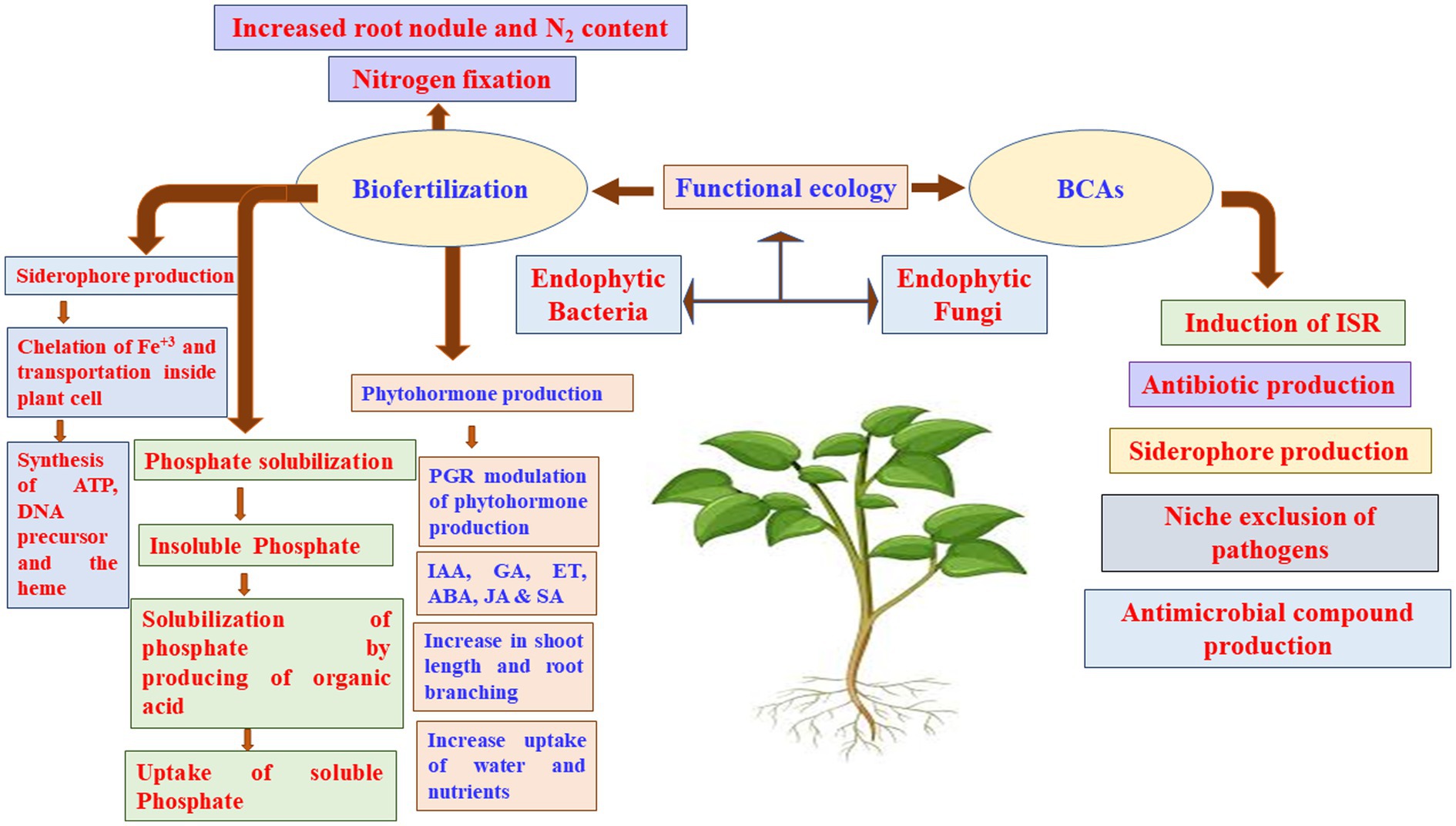
Figure 5. Dynamic microbial ecologies. ABA, abscisic acid; BCA, biocontrol agents; ET, ethylene; GA, gibberellic acid; IAA, indole acetic acid; ISR, induced systemic resistance; JA, jasmonic acid; PGR, plant growth regulators; SA, salicylic acid.
Competition in the rhizosphere
Co-existence of two living microorganisms occurs when a population of a particular microorganism strives to achieve something greater, such as space or food supply (Stirling, 2017). Pathogenic and non-pathogenic microorganisms compete for food and resources in the rhizosphere. It has been known for a long time that non-pathogenic plant-associated bacteria are usually protected by colonizing plants and, as a result, this debilitate the limited available substrates and prevent the spread of the pathogens. The abilities of any microorganism to compete with others for essential nutrients and exudates secreted by the plant roots and their capability of colonizing into the root surface of host plants are termed rhizosphere competence. In the rhizosphere, the beneficial interactions between plants and microorganisms can regularly occur; thus, promoting growth and/or enhancing tolerance to biotic and abiotic stresses in plants (Zamioudis and Pieterse, 2012).
Rhizosphere competence can successfully establish microbial communities on or near the plant roots. Plant root colonization by PGPM can protect plants against pathogens and promote plant growth, and chemotaxis to root exudates is considered as an essential prerequisite for efficient root colonization (de Weert and Bloemberg, 2006). Microbial community in the rhizosphere is found to be important shortly after planting, but regularly decreases during the cropping season (Weller, 2007). Adesina et al. (2009) have reported in vitro antagonism of 15 Pseudomonas strains to R. solani in the rhizosphere. Only Pseudomonas jessenii RU47 has been effective to bottom rot disease on lettuce caused by R. solani. Tryptophan can stimulate the growth of adventitious roots and root hairs of the IAA-producing B. subtilis SRB28, which can colonize root tissues in sorghum, produce microcolonies, and persist in the rhizosphere (Das et al., 2010).
Rhizosphere microorganisms promoting plant growth, represent a wide range of species. PGPM are categorized according to their ability to colonize roots, survive, increase their numbers in the microhabitats on the root surface, compete with indigenous microorganisms, and increase resistance in host crops (Gamalero et al., 2004). PGPM can not only promote plant development, but also they are often used as BCAs to suppress plant diseases. The plant-associated Bacillus, Pseudomonas, Lactobacillus and actinobacteria strains are used as biofertilizers and BCAs in agriculture (Borriss, 2011; Sivasakthi et al., 2014; Lamont et al., 2017; Shivlata and Satyanarayana, 2017). Furthermore, Acetobacter, Serratia, Azospirillum, Paenibacillus Burkholderia, Herbaspirillum, and Rhodococcus can also enhance growth in crop plants (Babalola, 2010). Chakraborty et al. (2013) reported that a number of PGPR traits, such as production of siderophore, solubilization of phosphate, synthesis of IAA, and antagonism against fungal pathogens, were found to stimulate growth in tea plants. This has been linked with an increase in the number of shoots and leaves under greenhouse and field conditions. In general, soils with active microbial ecosystems and high organic matter require less fertilizer than soils without any microorganisms (Bender et al., 2016).
Biofertilizers made from microorganisms that help plants obtain their nutrients can colonize plant roots, to solubilize P, produce siderophore and HCN, and fix N2 (Figure 5; Pii et al., 2015; El-Sobky et al., 2022). N2 fixation by PGPR provides a considerable amount of N to the farming systems worldwide, with estimations ranging from 20 to 22 Tg N annually (Herridge et al., 2008), which may reach in some years to up to 40 Tg N (Galloway et al., 2008). Moreover, it has been reported that the biological N2 fixation may provide the African countries approximately 12 Tg N year−1 (Elrys et al., 2019b, 2020b). Crop yields might be limited by other nutritional elements, such as Fe and Zn. Similar to P, Fe is highly abundant in soils; yet, it is not available to plants in most cases. The synthesis of organic acids or siderophores by various PGPR strains increases Fe accessibility (Ahmed and Holmstrom, 2014).
Auxins are produced by a variety of PGPR (Gupta et al., 2015) that is involved in plant growth and development (Jha and Saraf, 2015) and plant architecture (Vacheron et al., 2013). The auxin, IAA, produced by PGPR has received much of attention. It is highly involved during PGPR-plant interactions (Afzal et al., 2015). Auxin-producing PGPR have been reported to cause transcriptional alterations in the hormone levels, resistance/tolerance to biotic/abiotic stress, and regulation of cell wall-linked genes (Spaepen et al., 2014). IAA may also increase root length (Hong et al., 1991), enhance root biomass, while reducing the size and density of stomata (Llorente et al., 2016). Plant growth and development can also be stimulated by the induction of auxin-response genes (Ruzzi and Aroca, 2015).
In addition, PGPRs can produce GA and CKs (Gupta et al., 2015), although the exact process remains unknown (Kang et al., 2009). A limited number of PGPR strains can produce huge amounts of GA; thus, significantly increase the shoot growth in plants (Jha and Saraf, 2015). Exudates are expected to contain organic acids, sugars, and amino acids, which are highly abundant in the cytoplasm of plants, but low quantities of complex secondary metabolites, including flavonoids, terpenes, and phenolic substances, which may attract certain rhizosphere microorganisms (Musilova et al., 2016). Plant health and physiology could be improved due to PGPR colonization of roots, resulting in more seeds and blooms (Kumar et al., 2016). According to Nivedhitha et al. (2008), actinobacteria isolated from the rhizosphere of bamboo was found to be capable to suppress the fungal pathogen, Fusarium sp., while boosting plant growth and development. Harzianic acid produced by T. harzianum not only promoted plant growth, but also showed antifungal effects against Pythium irregulare, Sclerotinia sclerotiorum, and R. solani, even at very low doses (Vinale et al., 2009). MBCA are important to the advancement and improvement of plant growth development, as well as the prevention of the attack of plant pathogens.
Future perspectives
Biological control management is one of the most promising applications for sustainable agriculture. It is a proven to be eco-friendly agricultural pest control approach. This strategy uses living microorganisms to reduce the pest populations in a conservative, dependable, and ecologically amicable manner. In the developed countries, biological control is a remarkable tool to achieve sustainable, less expensive, and safe pest control management; thus, offering benefits to breeders and consumers when compared to synthetic (chemical) pest management. This review has provided an overview of antagonistic modes of action of MBCA, which are regarded as practical substitutions to synthetic fungicides as well as stimulation of plant growth and development for post-harvest purposes. Researchers working in the field of MBCA must anticipate new and distinct questions, in order to provide solutions that help in the development of novel biocontrol technologies/applications. Bioinformatics, molecular biology, analytical chemistry, and biostatistics have also shed lights on new research areas aimed at defining the MBCA-pathogen-plant interaction (Spadaro and Gullino, 2005).
One should not neglect the environmental conditions that also play a crucial role in the process of antagonism and the mode(s) of action of MBCA. The following conditions should be taken into consideration, when researchers isolate, identify and characterize a MBCA strain:
1. The spread of the infection associated with nematodes, fungi, and bacteria, as well as the potential antagonists in the micro- and macro-environment of the interaction.
2. The best conditions for the application of BCA.
3. The reaction of MBCA to the local communities and to various management strategies.
4. The limiting factors of effective colonization and articulation of biological control characteristics.
5. The plant components and dynamics that induce host defense.
Conclusion
Many crops are affected by various pathogens. PGPM of pests and diseases in crops are generally regarded as a sustainable alternative for conventional chemical plant protection. These PGPR and PGPF acting as MBCAs are a safe, effective, and environmentally friendly form of pest management that do not harm the environment or the human health. PGPR/PGPF are antagonistic microorganisms that could be exploited as biopesticides and biofertilizers for better plant health and growth improvement. Adoption of PGPR/PGPF-based biopesticides/biofertilizers on a commercial scale may substantially contribute to sustainable agriculture and safe environment. This review has provided an overview on the research related to PGPMs, their benefits and effects as potential bioinoculants for plant growth and biological control. The increased use of PGPMs requires the achievement of accurate selection of beneficial PGPR/PGPF strains and consortia, the mechanisms underlying PGPM-plant interactions, and the ability to prepare for future agricultural challenges.
Author contributions
All authors listed have made a substantial, direct, and intellectual contribution to the work and approved it for publication.
Funding
This project was funded by Abu Dhabi Education and Knowledge (Grant #: 21S105) to KE-T and Khalifa Center for Genetic Engineering and Biotechnology-UAEU (Grant #: 31R286) to SA.
Acknowledgments
KE-T woiuld like to thank the library at Murdoch University, Australia, for the valuable online resources and comprehensive databases.
Conflict of interest
The authors declare that the research was conducted in the absence of any commercial or financial relationships that could be construed as a potential conflict of interest.
Publisher’s note
All claims expressed in this article are solely those of the authors and do not necessarily represent those of their affiliated organizations, or those of the publisher, the editors and the reviewers. Any product that may be evaluated in this article, or claim that may be made by its manufacturer, is not guaranteed or endorsed by the publisher.
References
Abbas, R., Rasul, S., Aslam, K., Baber, M., Shahid, M., Mubeen, F., et al. (2019). Halotolerant PGPR: a hope for cultivation of saline soils. J. King Saud Univ. Sci. 31, 1195–1201. doi: 10.1016/j.jksus.2019.02.019
Abbasi, M. W., Ahmed, N., Zaki, M. J., Shaukat, S. S., and Khan, D. (2014). Potential of Bacillus species against Meloidogyne javanica parasitizing eggplant (Solanum melongena L.) and induced biochemical changes. Plant Soil 375, 159–173. doi: 10.1007/s11104-013-1931-6
Abd El-Mageed, T. A., Rady, M. M., Taha, R. S., Abd El Azeam, S., Simpson, C. R., and Semida, W. M. (2020). Effects of integrated use of residual sulfur-enhanced biochar with effective microorganisms on soil properties, plant growth and short-term productivity of Capsicum annuum under salt stress. Sci. Hortic. 261:108930. doi: 10.1016/j.scienta.2019.108930
Abriouel, H., Franz, C. M. A. P., Omar, N. B., and Gálvez, A. (2011). Diversity and applications of Bacillus bacteriocins. FEMS Microbiol. Rev. 35, 201–232. doi: 10.1111/j.1574-6976.2010.00244.x
AbuQamar, S. F., Moustafa, K., and Tran, L. S. (2017). Mechanisms and strategies of plant defense against Botrytis cinerea. Crit. Rev. Biotechnol. 37, 262–274. doi: 10.1080/07388551.2016.1271767
Adebola, M. O., and Amadi, J. E. (2010). Screening three Aspergillus species for antagonistic activities against the cocoa black pod organism (Phytophthora palmivora). Agric. Biol. J. North Am. 1, 362–365. doi: 10.5251/abjna.2010.1.3.362.365
Adesina, M. F., Grosch, R., Lembke, A., Vatchev, T. D., and Smalla, K. (2009). In vitro antagonists of Rhizoctonia solani tested on lettuce: rhizosphere competence, biocontrol efficiency and rhizosphere microbial community response. FEMS Microbiol. Ecol. 69, 62–74. doi: 10.1111/j.1574-6941.2009.00685.x
Afzal, I., Shinwari, Z. K., and Iqrar, I. (2015). Selective isolation and characterization of agriculturally beneficial endophytic bacteria from wild hemp using canola. Pak. J. Bot. 47, 1999–2008.
Agarwal, T., Malhotra, A., Trivedi, P. C., and Biyani, M. (2011). Biocontrol potential of Gliocladium virens against fungal pathogens isolated from chickpea, lentil and black gram seeds. Int. J. Agric. Technol. 7, 1833–1839.
Agbor, C., Djueani, A. C., Muyang, R. F., and Mbouobda, H. D. (2021). Rhizopus spp. promoting and inducing late blight (Phytophthora infestans) resistance in Solanum lycopersicum L. Annu. Res. Rev. Biol. 36, 1–13. doi: 10.9734/arrb/2021/v36i230338
Ahmed, E., and Holmstrom, S. J. (2014). Siderophores in environmental research: roles and applications. J. Microbial. Biotechnol. 7, 196–208. doi: 10.1111/1751-7915.12117
Al Hamad, B. M., Al Raish, S. M., Ramadan, G. A., Saeed, E. E., Alameri, S. S. A., Al Senaani, S. S., et al. (2021). Effectiveness of augmentative biological control of Streptomyces griseorubens UAE2 depends on 1-aminocyclopropane-1-carboxylic acid deaminase activity against Neoscytalidium dimidiatum. J. Fungi. 7:885. doi: 10.3390/jof7110885
Al Raish, S. M., Saeed, E. E., Alyafei, D. M., El-Tarabily, K. A., and AbuQamar, S. F. (2021). Evaluation of streptomycete actinobacterial isolates as biocontrol agents against royal poinciana stem canker disease caused by the fungal pathogen Neoscytalidium dimidiatum. Biol. Control 164:104783. doi: 10.1016/j.biocontrol.2021.104783
Alam, S. S., Sakamoto, K., Amemiya, Y., and Inubushi, K. (2011). Biocontrol efficiency of Fusarium wilt diseases by a root-colonizing fungus Penicillium sp. Soil Sci. Plant Nutr. 57, 204–212. doi: 10.1080/00380768.2011.564996
Alblooshi, A. A., Purayil, G. P., Saeed, E. E., Ramadan, G. A., Tariq, S., Altaee, A. S., et al. (2022). Biocontrol potential of endophytic actinobacteria against Fusarium solani, the causal agent of sudden decline syndrome on date palm in the UAE. J. Fungi. 8:8. doi: 10.3390/jof8010008
Al-Hussini, H. S., Al-Rawahi, A. Y., Al-Marhoon, A. A., Al-Abri, S. A., Al-Mahmooli, I. H., Al-Sadi, A. M., et al. (2019). Biological control of damping-off of tomato caused by Pythium aphanidermatum by using native antagonistic rhizobacteria isolated from Omani soil. J. Plant Pathol. 101, 315–322. doi: 10.1007/s42161-018-0184-x
Alwahshi, K. J., Purayil, G. P., Saeed, E. E., Abufarajallah, H. A., Aldhaheri, S. J., AbuQamar, S. F., et al. (2022). The ACC deaminase-producing Streptomyces violaceoruber UAE1 can provide further protection from sudden decline syndrome on date palm. Front. Plant Sci. 13:904166. doi: 10.3389/fpls.2022.904166
Anastasiadis, I. A., Giannakou, I. O., Prophetou-Athanasiadou, D. A., and Gowen, S. R. (2008). The combined effect of the application of a biocontrol agent Paecilomyces lilacinus, with various practices for the control of root-knot nematodes. Crop Prot. 27, 352–361. doi: 10.1016/j.cropro.2007.06.008
Anitha, R., and Murugesan, K. (2005). Production of gliotoxin on natural substrates by Trichoderma virens. J. Basic Microbiol. 45, 12–19. doi: 10.1002/jobm.200410451
Antoun, H., and Kloepper, J. (2001). “Plant growth promoting rhizobacteria,” in Encyclopedia of genetics. eds. S. Brenner and J. H. Miller (New York: Academic Press), 1477–1480.
Arseneault, T., and Filion, M. (2017). Biocontrol through antibiosis: exploring the role played by subinhibitory concentrations of antibiotics in soil and their impact on plant pathogens. Can. J. Plant Pathol. 39, 267–274. doi: 10.1080/07060661.2017.1354335
Arya, R. (2011). Effect of culture filtrates of Trichoderma viridae and Trichothecium roseum grown on different carbon and nitrogen sources on hatching and mortality of root-knot nematode (Meloidogyne incognita). Indian J. Nematol. 41, 62–70.
Ashajyothia, M., Kumara, A., Sheorana, N., Ganesana, P., Gogoia, R., Subbaiyanb, G. K., et al. (2020). Black pepper (Piper nigrum L.) associated endophytic Pseudomonas putida BP25 alters root phenotype and induces defense in rice (Oryza sativa L.) against blast disease incited by Magnaporthe oryzae. Biol. Control 143. doi: 10.1016/j.biocontrol.2019.104181
Atkins, S. D., Clark, I. M., Pande, S., Hirsch, P. R., and Kerry, B. K. (2005). The use of real-time PCR and species-specific primers for the identification and monitoring of Paecilomyces lilacinus. FEMS Microbiol. Ecol. 51, 257–264. doi: 10.1016/j.femsec.2004.09.002
Babalola, O. O. (2010). Beneficial bacteria of agricultural importance. Biotechnol. Lett. 32, 1559–1570. doi: 10.1007/s10529-010-0347-0
Barratt, B. I. P., Moran, V. C., Bigler, F., and van Lenteren, J. C. (2018). The status of biological control and recommendations for improving uptake for the future. Biol. Control 63, 155–167. doi: 10.1007/s10526-017-9831-y
Bashan, Y., de Bashan, L. E., Prabhu, S. R., and Hernandez, J. -P. (2014). Advances in plant growth-promoting bacterial inoculant technology: formulations and practical perspectives (1998–2013). Plant Soil 378, 1–33. doi: 10.1007/s11104-013-1956-x
Begum, M., Lees, E., Ampt, P., and Mansfield, S. (2017). Development of Australian commercial producers of invertebrate biological control agents from 1971 to 2014. Biol. Control 62, 525–533. doi: 10.1007/s10526-017-9822-z
Bender, S. F., Wagg, C., and Van Der Heijden, M. G. A. (2016). An underground revolution: biodiversity and soil ecological engineering for agricultural sustainability. Trends Ecol. Evol. 31, 440–452. doi: 10.1016/j.tree.2016.02.016
Beneduzi, A., Ambrosini, A., and Passaglia, L. M. P. (2012). Plant growth promoting rhizobacteria (PGPR): their potential as antagonists and biocontrol agents. Genet. Mol. Biol. 35, 1044–1051. doi: 10.1590/S1415-47572012000600020
Berger, B., Patz, S., Ruppel, S., Dietel, K., Faetke, S., Junge, H., et al. (2018). Successful formulation and application of plant growth-promoting Kosakonia radicincitans in maize cultivation. Biomed. Res. Int. 2018:6439481. doi: 10.1155/2018/6439481
Berry, C. L., Nandi, M., Manuel, J., Brassinga, A. K. C., Fernando, W. G. D., Loewen, P. C., et al. (2014). Characterization of the Pseudomonas sp. DF41 quorum sensing locus and its role in fungal antagonism. Biol. Control 69, 82–89. doi: 10.1016/j.biocontrol.2013.11.005
Bhat, M. A., Kumar, V., Bhat, M. A., Wani, I. A., Dar, F. L., Farooq, I., et al. (2020). Mechanistic insights of the interaction of plant growth-promoting rhizobacteria (PGPR) with plant roots toward enhancing plant productivity by alleviating salinity stress. Front. Microbiol. 11:1952. doi: 10.3389/fmicb.2020.01952
Bhattacharyya, P. N., and Jha, D. K. (2012). Plant growth-promoting rhizobacteria (PGPR): emergence in agriculture. World J. Microbiol. Biotechnol. 28, 1327–1350. doi: 10.1007/s11274-011-0979-9
Bilesky-José, N., Maruyama, C., Germano-Costa, T., Campos, E., Carvalho, L., Grillo, R., et al. (2021). Biogenic α-Fe2O3 nanoparticles enhance the biological activity of Trichoderma against the plant pathogen Sclerotinia sclerotiorum. ACS Sustain. Chem. Eng. 9, 1669–1683. doi: 10.1021/acssuschemeng.0c07349
Bokhari, F. M. (2009). Efficacy of some Trichoderma species in the control of Rotylenchulus reniformis and Meloidogyne javanica. Arch. Phytopathol. Plant Prot. 42, 361–369. doi: 10.1080/03235400601070520
Bonaterra, A., Badosa, E., Cabrefiga, J., Frances, J., and Montesinos, E. (2012). Prospects and limitations of microbial pesticides for control of bacterial and fungal pomefruit tree diseases. Trees 26, 215–226. doi: 10.1007/s00468-011-0626-y
Bontempo, A. F., Lopes, E. A., Fernandes, R. H., De Freitas, L. G., and Dallemole-Giaretta, R. (2017). Dose-response effect of Pochonia chlamydosporia against Meloidogyne incognita on carrot under field conditions. Revista Caatinga Mossoró. 30, 258–262. doi: 10.1590/1983-21252017v30n129rc
Borriss, R. (2011). “Use of plant-associated Bacillus strains as biofertilizers and biocontrol agents in agriculture,” in Bacteria in agrobiology: Plant growth responses. ed. D. K. Maheshwari (Berlin: Springer), 41–76.
Carrasco-Espinosa, K., García-Cabrera, R. I., Bedoya-López, A., Trujillo-Roldán, M. A., and Valdez-Cruz, N. A. (2015). Positive effect of reduced aeration rate on growth and stereospecificity of dl-malic acid consumption by Azospirillum brasilense: improving the shelf life of a liquid inoculant formulation. J. Biotechnol. 195, 74–81. doi: 10.1016/j.jbiotec.2014.12.020
Chakraborty, U., Chakraborty, B. N., Chakraborty, A. P., Sunar, K., and Dey, P. L. (2013). Plant growth promoting rhizobacteria mediated improvement of health status of tea plants. Indian J. Biotechnol. 12, 20–31.
Chakruno, P., Banik, S., and Sumi, K. (2022). “Important diseases of tea (Camellia sinensis L.) and their integrated management,” in Diseases of Horticultural Crops: Diagnosis and Management. eds. J. N. Srivastava and A. K. Singh (New York: Apple Academic Press), 119–138.
Chaloner, T. M., Gurr, S. J., and Bebber, D. P. (2021). Plant pathogen infection risk tracks global crop yields under climate change. Nat. Clim. Chang. 11, 710–715. doi: 10.1038/s41558-021-01104-8
Cheng, X., Ji, X., Li, J., Qi, W., Ge, Y., and Qiao, K. (2019). Characterization of antagonistic Bacillus methylotrophicus isolated from rhizosphere and its biocontrol effects on maize stalk rot. Phytopathology 109, 571–581. doi: 10.1094/phyto-07-18-0220-r
Chiranjeevi, N., Kumar, M. R., Padmodaya, B., Venkateswarlu, N. C., Sudhakar, P., Devi, R. S. J., et al. (2021). Studies on extraction, evaluation of crude metabolite extract from endophytic Bacillus subtillis and its mechanistic effect on chickpea dry root rot causing pathogen Rhizoctonia bataticola (Taub.) Butler. Pharma Innov. 10, 898–905.
Choudhary, D. K., Sharma, K. P., and Gaur, R. K. (2011). Biotechnological perspectives of microbes in agro-ecosystems. Biotechnol. Lett. 33, 1905–1910. doi: 10.1007/s10529-011-0662-0
Chowdhury, S. P., Hartmann, A., Gao, X., and Borriss, R. (2015). Biocontrol mechanism by root-associated Bacillus amyloliquefaciens FZB42: a review. Front. Microbiol. 6:780. doi: 10.3389/fmicb.2015.00780
Compant, S., Clément, C., and Sessitsch, A. (2010). Plant growth-promoting bacteria in the rhizo and endosphere of plants: their role, colonization, mechanisms involved and prospects for utilization. Soil Biol. Biochem. 42, 669–678. doi: 10.1016/j.soilbio.2009.11.024
Compant, S., Duffy, B., Nowak, J., Clément, C., and Barka, E. A. (2005). Use of plant growth-promoting bacteria for biocontrol of plant diseases: principles, mechanisms of action, and future prospects. Appl. Environ. Microbiol. 71, 4951–4959. doi: 10.1128/AEM.71.9.4951-4959.2005
Das, S. N., Sarma, P. V., Neeraja, C., Malati, N., and Podile, A. R. (2010). Members of gamma proteobacteria and bacilli represent the culturable diversity of chitinolytic bacteria in chitin-enriched soils. World J. Microbiol. Biotechnol. 26, 1875–1881. doi: 10.1007/s11274-010-0369-8
De la Cruz-Quiroz, R., Roussos, S., Rodríguez-Herrera, R., Hernandez-Castillo, D., and Aguilar, C. N. (2018). Growth inhibition of Colletotrichum gloeosporioides and Phytophthora capsici by native Mexican Trichoderma strains. Karbala Int. J. Mod. Sci. 4, 237–243. doi: 10.1016/j.kijoms.2018.03.002
de Weert, S., and Bloemberg, G. V. (2006). “Rhizosphere competence and the role of root colonization in biocontrol” in Plant-Associated Bacteria. ed. S. S. Gnanamanickam (Dordrecht: Springer), 317–333.
Di Francesco, A., and Baraldi, E. (2021). How siderophore production can influence the biocontrol activity of Aureobasidium pullulans against Monilinia laxa on peaches. Biol. Control 152:104456. doi: 10.1016/j.biocontrol.2020.104456
Donley, N. (2019). The USA lags behind other agricultural nations in banning harmful pesticides. Environ. Health 18:44. doi: 10.1186/s12940-019-0488-0
Duffy, B., Schouten, A., and Raaijmakers, J. M. (2003). Pathogen self-defense: mechanisms to counteract microbial antagonism. Annu. Rev. Phytopathol. 41, 501–538. doi: 10.1146/annurev.phyto.41.052002.095606
Dukarea, A., and Paulb, S. (2021). Biological control of Fusarium wilt and growth promotion in pigeon pea (Cajanus cajan) by antagonistic rhizobacteria, displaying multiple modes of pathogen inhibition. Rhizosphere 17:100278. doi: 10.1016/j.rhisph.2020.100278
Elnahal, A. S., El-Saadony, M. T., Saad, A. M., Desoky, E. S. M., El-Tahan, A. M., Rady, M. M., et al. (2022). The use of microbial inoculants for biological control, plant growth promotion, and sustainable agriculture: a review. Eur. J. Plant Pathol. 162, 759–792. doi: 10.1007/s10658-021-02393-7
Elrys, A. S., Abdel-Fattah, M. K., Raza, S., Chen, Z., and Zhou, J. (2019b). Spatial trends in the budget of nitrogen flows in the African agro-food system over the past five decades. Environ. Res. Lett. 14:124091. doi: 10.1088/1748-9326/ab5d9e
Elrys, A. S., Abdo, A. I. E., Abdel-Hamed, E. M., and Desoky, E. S. M. (2020a). Integrative application of licorice root extract or lipoic acid with fulvic acid improves wheat production and defenses under salt stress conditions. Ecotoxicol. Environ. Saf. 190:110144. doi: 10.1016/j.ecoenv.2019.110144
Elrys, A. S., Desoky, E.-S. M., Abo El-Maati, M. F., Elnahal, A. S., Abdo, A. I., Raza, S., et al. (2019a). Can secondary metabolites extracted from moringa seeds suppress ammonia oxidizers to increase nitrogen use efficiency and reduce nitrate contamination in potato tubers? Ecotoxicol. Environ. Saf. 185:109689. doi: 10.1016/j.ecoenv.2019.109689
Elrys, A. S., Metwally, M. S., Raza, S., Alnaimy, M. A., Shaheen, S. M., Chen, Z., et al. (2020b). How much nitrogen does Africa need to feed itself by 2050? J. Environ. Manage. 268:110488. doi: 10.1016/j.jenvman.2020.110488
El-Sobky, E. -S. E. A., Taha, A. E., El-Sharnouby, M., Sayed, S. M., and Elrys, A. S. (2022). Zinc-biochemical co-fertilization improves rice performance and reduces nutrient surplus under semi-arid environmental conditions. Saudi J. Biol. Sci. 29, 653–1667. doi: 10.1016/j.sjbs.2021.10.066
Esfahani, N. M., and Pour, B. A. (2006). The effects of Paecilomyces lilacinus on the pathogenesis of Meloidogyne javanica and tomato plant growth. Iran Agric. Res. 24, 67–76.
Faizal Azizi, M. M., and Lau, H. Y. (2022). Advanced diagnostic approaches developed for the global menace of rice diseases: a review. Can. J. Plant Pathol. 1–25, 1–25. doi: 10.1080/07060661.2022.2053588
Fan, D., Subramanian, S., and Smith, D. L. (2020). Plant endophytes promote growth and alleviate salt stress in Arabidopsis thaliana. Sci. Rep. 10:12740. doi: 10.1038/s41598-020-69713-5
Farhat, H., Urooj, F., Shafique, H. A., Sultana, V., and Ehteshamul-Haque, S. (2017). Rhizobia suppress the root knot nematode and root rotting fungi on mungbean. Int. J. Biol. Res. 5, 71–75.
Ferro, H. M., Souza, R., Lelis, F., Vieira, M., Silva, J. C. P. D., and Medeiros, F. H. V. D. (2020). Bacteria for cotton plant protection: disease control, crop yield and fiber quality. Rev. Caatinga 33, 43–53. doi: 10.1590/1983-21252020v33n105rc
Fones, H. N., Bebber, D. P., Chaloner, T. M., Kay, W. T., Steinberg, G., Gurr, S. J., et al. (2020). Threats to global food security from emerging fungal and oomycete crop pathogens. Nat. Food. 1, 332–342. doi:10.1038/ s43016-020-0075-0
Fu, B., Olawole, O., and Beattie, G. A. (2021). Biological control and microbial ecology draft genome sequence data of Glutamicibacter sp. FBE-19, a bacterium antagonistic to the plant pathogen Erwinia tracheiphila. Phytopathology 111, 765–768. doi: 10.1094/PHYTO-09-20-0380-A
Galloway, J. N., Townsend, A. R., Erisman, J. W., Bekunda, M., Cai, Z., Freney, J. R., et al. (2008). Transformation of the nitrogen cycle: recent trends, questions, and potential solutions. Science 320, 889–892. doi: 10.1126/science.1136674
Gamalero, E., Lingua, G., Capri, F. G., Fusconi, A., Berta, G., and Lemanceau, P. (2004). Colonization pattern of primary tomato roots by Pseudomonas fluorescens A6RI characterized by dilution plating, flow cytometry, fluorescence, confocal and scanning electron microscopy. FEMS Microbiol. Ecol. 48, 79–87. doi: 10.1016/j.femsec.2003.12.012
Gao, Q. -M., Zhu, S., Kachroo, P., and Kachroo, A. (2015). Signal regulators of systemic acquired resistance. Front. Plant Sci. 6:228. doi: 10.3389/fpls.2015.00228
Gaziea-Soliman, M., Ameen, H. H., and El-kelany, U. S. (2017). Effect of treatment time on biocontrol efficacy of Bacillus amyloliquefaciens, Lysinibacillus sphaericus and their Fusants against root-knot nematode Meloidogyne incognita infecting tomato plants. Middle East J. Agric. Res. 6, 369–375.
Ghazanfar, M. U., Hussan, M., Hamid, M. I., and Ansari, S. U. (2016). Utilization of biological control agents for the management of postharvest pathogens of tomato. Pak. J. Bot. 48, 2093–2100.
Gilden, R. C., Huffling, K., and Sattler, B. (2010). Pesticides and health risks. J. Obstet. Gynecol. Neonatal Nurs. 39, 103–110. doi: 10.1111/j.1552-6909.2009.01092.x
Girlanda, M., Perotto, S., Mönne-Loccoz, Y., Bergero, R., Lazzari, A., Défago, G., et al. (2001). Impact of biocontrol Pseudomonas fluorescens CHA0 and a genetically modified derivative on the diversity of culturable fungi in the cucumber rhizosphere. Appl. Environ. Microbiol. 67, 1851–1864. doi: 10.1128/AEM.67.4.1851-1864.2001
Giron, D., Frago, E., Glevarec, G., Pieterse, C. M., and Dicke, M. (2013). Cytokinins as key regulators in plant–microbe–insect interactions: connecting plant growth and defence. Funct. Ecol. 27, 599–609. doi: 10.1111/1365-2435.12042
Glare, T., Caradus, J., Gelernter, W., Jackson, T., Keyhani, N., Kohl, J., et al. (2012). Have biopesticides come of age? Trends Biotechnol. 30, 250–258. doi: 10.1016/j.tibtech.2012.01.003T
Glick, B. (1995). The enhancement of plant growth by free-living bacteria. Can. J. Microbiol. 41, 109–117. doi: 10.1139/m95-015
Glick, B. R. (2012). Plant growth-promoting bacteria: mechanisms and applications. Scientifica 2012:963401. doi: 10.6064/2012/963401
Goh, C. H., Veliz Vallejos, D. F., Nicotra, A. B., and Mathesius, U. (2013). The impact of beneficial plant-associated microbes on plant phenotypic plasticity. J. Chem. Ecol. 39, 826–839. doi: 10.1007/s10886-013-0326-8
Goswami, M., and Deka, S. (2020). Plant growth-promoting rhizobacteria—alleviators of abiotic stresses in soil: a review. Pedosphere 30, 40–61. doi: 10.1016/S1002-0160(19)60839-8
Gouda, S., Kerry, R. G., Das, G., Paramithiotis, S., Shin, H. -S., and Patra, J. K. (2018). Revitalization of plant growth promoting rhizobacteria for sustainable development in agriculture. Microbiol. Res. 206, 131–140. doi: 10.1016/j.micres.2017.08.016
Gowtham, H. G., Hariprasad, P., Nayak, S. C., and Niranjana, S. R. (2016). Application of rhizobacteria antagonistic to Fusarium oxysporum f. sp. lycopersici for the management of Fusarium wilt in tomato. Rhizosphere 2, 72–74. doi: 10.1016/j.rhisph.2016.07.008
Gupta, G., Parihar, S. S., Ahirwar, N. K., Snehi, S. K., and Singh, V. (2015). Plant growth promoting rhizobacteria (PGPR): current and future prospects for development of sustainable agriculture. J. Microb. Biochem. Technol. 7, 096–102. doi: 10.4172/1948-5948.1000188
Haas, D., and Keel, C. (2003). Regulation of antibiotic production in root colonizing Pseudomonas spp. and relevance for biological control of plant disease. Annu. Rev. Phytopathol. 41, 117–153. doi: 10.1146/annurev.phyto.41.052002.095656
Habiba, N. R., Ali, S. A., Sultana, V., Ara, J., and Ehteshamul-Haque, S. (2016). Evaluation of biocontrol potential of epiphytic fluorescent Pseudomonas associated with healthy fruits and vegetables against root rot and root knot pathogens of mungbean. Pak. J. Bot. 48, 1299–1303.
Hajek, A. E., Hurley, B. P., Kenis, M., Garnas, J. R., Bush, S. J., Wingfield, J. M. J., et al. (2016). Exotic biological control agents: a solution or contribution to arthropod invasions? Biol. Invasions 18, 953–969. doi: 10.1007/s10530-016-1075-8
Hanawi, M. J. (2016). Tagetes erecta with native isolates of Paecilomyces lilacinus and Trichoderma hamatum in controlling root-knot nematode Meloidogyne javanica on tomato. Int. J. Appl. Innov. Eng. Manag. 5, 81–88.
Harman, G. E., Howell, C. R., Viterbo, A., Chet, I., and Lorito, M. (2004). Trichoderma species—opportunistic, avirulent plant symbionts. Nat. Rev. Microbiol. 2, 43–56. doi: 10.1038/nrmicro797
Heimpel, G. E., Yang, Y., Hill, J. D., and Ragsdale, D. W. (2013). Environmental consequences of invasive species: greenhouse gas emissions of insecticide use and the role of biological control in reducing emissions. PLoS One 8:e72293. doi: 10.1371/journal.pone.0072293
Hernandez-Leon, R., Rojas-Solis, D., Contreras-Perez, M., Orozco-Mosqueda, M. C., Macias-Rodriguez, L. I., la Cruz, H. R. D., et al. (2015). Characterization of the antifungal and plant growth-promoting effects of diffusible and volatile organic compounds produced by Pseudomonas fluorescens strains. Biol. Control 81, 83–92. doi: 10.1016/j.biocontrol.2014.11.011
Herridge, D. F., Peoples, M. B., and Boddey, R. M. (2008). Global inputs of biological nitrogen fixation in agricultural systems. Plant Soil 311, 1–18. doi: 10.1007/s11104-008-9668-3
Hidayah, B. N., Khangura, R., and Dell, B. (2022). Biological control potential of Trichoderma species and bacterial antagonists against Sclerotinia sclerotiorum on canola in Western Australia. Int. J. Agric. Biol. 27, 215–227. doi: 10.17957/ijab/15.1919
Hong, Y. W., Glick, B. R., and Pasternak, J. J. (1991). Plant microbial interaction under gnotobiotic conditions: a scanning electron microscope study. Curr. Microbiol. 23, 111–114. doi: 10.1007/bf02092259
Hussain, M., Zouhar, M., and Ryšánek, P. (2017). Comparison between biological and chemical management of root-knot nematode, Meloidogyne hapla. Pak. J. Zool. 49, 205–210. doi: 10.17582/journal.pjz/2017.49.1.215.220
Ibiang, S. R., Usami, T., and Sakamoto, K. (2021). Reduction of Verticillium wilt in tomato by an arbuscular mycorrhizal fungus-Rhizophagus intraradices and an endophytic fungus-Penicillium pinophilum is cultivar dependent. Rhizosphere 20:100440. doi: 10.1016/j.rhisph.2021.100440
Inui Kishi, R. N., Galdiano Júnior, R. F., Val-Moraes, S. P., and Kishi, L. T. (2017). “Soil microbiome and their effects on nutrient management for plants,” in Probiotics in Agroecosystem. eds. V. Kumar, M. Kumar, S. Sharma, and R. Prasad (Singapore: Springer), 117–143.
Islam, S., Akanda, A. M., Prova, A., Islam, M. T., and Hossain, M. M. (2016). Isolation and identification of plant growth promoting rhizobacteria from cucumber rhizosphere and their effect on plant growth promotion and disease suppression. Front. Microbiol. 6:1360. doi: 10.3389/fmicb.2015.01360
Jha, C. K., and Saraf, M. (2015). Plant growth promoting rhizobacteria (PGPR): a review. E3 J. Agric. Res. Dev. 2, 108–119. doi: 10.13140/RG.2.1.5171.2164
Jha, S. N., Vishwakarma, R. K., Ahmad, T., Rai, A., and Dixit, A. K. (2015). Technical report on assessment of quantitative harvest and post-harvest losses of major crops and commodities in India. Ludhiana: ICAR All India Coordinated Research Project on Post-Harvest Technology, ICAR-CIPHET. doi: 10.13140/rg.2.1.3024.3924
Jisha, M. S., Linu, M. S., and Sreekumar, J. (2018). Induction of systemic resistance in chilli (Capsicum annuum L.) by Pseudomonas aeruginosa against anthracnose pathogen Colletotrichum capsici. J. Trop. Agric. 56, 153–166.
Jochum, M. D., McWilliams, K. L., Borrego, E. J., Kolomiets, M. V., Niu, G., Pierson, E. A., et al. (2019). Bioprospecting plant growth-promoting rhizobacteria that mitigate drought stress in grasses. Front. Microbiol. 10:2106. doi: 10.3389/fmicb.2019.02106
Jones, E. E., Rabeendran, N., and Stewart, A. (2014). Biocontrol of Sclerotinia sclerotiorum infection of cabbage by Coniothyrium minitans and Trichoderma spp. Biocontrol Sci. Tech. 24, 1363–1382. doi: 10.1080/09583157.2014.940847
Kakembo, D., and Lee, Y. H. (2019). Analysis of traits for biocontrol performance of Pseudomonas parafulva JBCS1880 against bacterial pustule in soybean plants. Biol. Control 134, 72–81. doi: 10.1016/j.biocontrol.2019.04.006
Kalele, D. N., Affokpon, A., Coosemans, J., and Kimenju, J. (2010). Suppression of root-knot nematodes in tomato and cucumber using biological control agents. Afr. J. Hoticultural. Sci. 3, 72–80.
Kamil, F. H., Saeed, E. E., El-Tarabily, K. A., and AbuQamar, S. F. (2018). Biological control of mango dieback disease caused by Lasiodiplodia theobromae using streptomycete and non-streptomycete actinobacteria in the United Arab Emirates. Front. Microbiol. 9:829. doi: 10.3389/fmicb.2018.00829
Kang, S. M., Joo, G. J., Hamayun, M., Na, C. I., Shin, D. H., Kim, H. Y., et al. (2009). Gibberellin production and phosphate solubilization by newly isolated strain of Acinetobacter calcoaceticus and its effect on plant growth. Biotechnol. Lett. 31, 277–281. doi: 10.1007/s10529-008-9867-2
Kang, S. W., Rahman, M. S., Kim, A. N., Lee, K. Y., Park, C. Y., Kerr, W. L., et al. (2017). Comparative study of the quality characteristics of defatted soy flour treated by supercritical carbon dioxide and organic solvent. J. Food Sci. Technol. 54, 2485–2493. doi: 10.1007/s13197-017-2691-8
Kavitha, P. G., Jonathan, E. L., and Sankari Meena, K. (2013). Induction of defence enzymes in tomato by plant growth promoting rhizobacteria, Pseudomonas fluorescens against root-knot nematode, Meloidogyne incognita. Indian J. Nematol. 43, 94–96.
Kazan, K., and Manners, J. M. (2009). Linking development to defense: auxin in plant–pathogen interactions. Trends Plant Sci. 14, 373–382. doi: 10.1016/j.tplants.2009.04.005
Kerry, B. R., and Hidalgo-Diaz, L. (2004). Application of Pochonia chlamydosporia in the integrated control of root-knot nematodes on organically grown vegetable crops in Cuba. Bull. IOBC/WPRS/SROP 27, 123–126.
Khafagi, E. Y., El-Syed, A., and Elwan, S. E. (2020). Controlling cabbage Fusarium wilt (yellows) using topsin M and some commercial biofertilizer products. Zagazig J. Agric. Res. 47, 519–530. doi: 10.21608/zjar.2020.94492
Khalili, E., Sadravi, M., Naeimi, S., and Khorsavi, V. (2012). Biological control of rice brown spot with native isolates of three Trichoderma species. Braz. J. Microbiol. 43, 297–305. doi: 10.1590/S1517-838220120001000035
Khan, A., Shaukat, S. S., Islam, S., and Khan, A. (2012). Evaluation of fluorescent Pseudomonas isolates for their activity against some plant-parasitic nematodes. Am. Eurasian J. Agric. Environ. Sci. 12, 1496–1506. doi: 10.5829/idosi.aejaes.2012.12.11.1841
Khan, A., Tariq, M., Asif, M., Khan, F., Ansari, T., and Siddiqui, M. A. (2019). Integrated management of Meloidogyne incognita infecting Vigna radiata L. using biocontrol agent Purpureocillium lilacinum. Trends Appl. Sci. Res. 14, 119–124. doi: 10.3923/tasr.2019.119.124
Khatun, A., Farhana, T., Sabir, A. A., Islam, S., West, H. M., Rahman, M., et al. (2018). Pseudomonas and Burkholderia inhibit growth and asexual development of Phytophthora capsici. Z. Naturforsch. C J. Biosci. 73, 123–135. doi: 10.1515/znc-2017-0065
Kim, Y. S., Song, J. G., Lee, I. K., Yeo, W. H., and Yun, B. S. (2013). Bacillus sp. BS061 suppresses powdery mildew and gray mold. Mycobiology 41, 108–111. doi: 10.5941/myco.2013.41.2.108
Kiriga, A. W., Haukeland, S., Kariuki, G. M., Coyne, D. L., and Beek, N. V. (2018). Effect of Trichoderma spp. and Purpureocillium lilacinum on Meloidogyne javanica in commercial pineapple production in Kenya. Biol. Control 119, 27–32. doi: 10.1016/j.biocontrol.2018.01.005
Kiss, L. (2003). A review of fungal antagonists of powdery mildews and their potential as biocontrol agents. Pest Manag. Sci. 59, 475–483. doi: 10.1002/ps.689
Kloepper, J. W., Leong, J., Teintze, M., and Schroth, M. N. (1980). Enhanced plant growth by siderophores produced by plant growth-promoting rhizobacteria. Nature 286, 885–886. doi: 10.1038/286885a0
Köhl, J., Kolnaar, R., and Ravensberg, W. J. (2019). Mode of action of microbial biological control agents against plant diseases: relevance beyond efficacy. Front. Plant Sci. 10:845. doi: 10.3389/fpls.2019.00845
Kumar, S., Chauhan, P. S., Agrawal, L., Raj, R., Srivastava, A., Gupta, S., et al. (2016). Paenibacillus lentimorbus inoculation enhances tobacco growth and extenuates the virulence of cucumber mosaic virus. PLoS One 11:e0149980. doi: 10.1371/journal.pone.0149980
Kumar, V., Singh, A. U., and Jain, R. K. (2012). Comparative efficacy of bioagents as seed treatment for management of Meloidogyne incognita infecting okra. Nematologia Mediterr. 40, 209–211.
Lamont, J. R., Wilkins, O., Bywater-Ekegard, M., and Smith, D. L. (2017). From yogurt to yield: potential applications of lactic acid bacteria in plant production. Soil Biol. Biochem. 111, 1–9. doi: 10.1016/j.soilbio.2017.03.015
Lee, Y. S., and Kim, K. Y. (2016). Antagonistic potential of Bacillus pumilus L1 against root-knot nematode, Meloidogyne arenaria. J. Phytopathol. 164, 29–39. doi: 10.1111/jph.12421
Lee, S. K., Lur, H. S., Lo, K. J., Cheng, K. C., Chuang, C. C., Tang, S. J., et al. (2016). Evaluation of the effects of different liquid inoculant formulations on the survival and plant-growth-promoting efficiency of Rhodopseudomonas palustris strain PS3. Appl. Microbiol. Biotechnol. 100, 7977–7987. doi: 10.1007/s00253-016-7582-9
Leontidou, K., Genitsaris, S., Papadopoulou, A., Kamou, N., Bosmali, I., Matsi, T., et al. (2020). Plant growth promoting rhizobacteria isolated from halophytes and drought-tolerant plants: genomic characterization and exploration of phyto-beneficial traits. Sci. Rep. 10:14857. doi: 10.1038/s41598-020-71652-0
Li, Y., Feng, X., Wang, X., Zheng, L., and Liu, H. (2020). Inhibitory effects of Bacillus licheniformis BL06 on Phytophthora capsici in pepper by multiple modes of action. Biol. Control 144:104210. doi: 10.1016/j.biocontrol.2020.104210
Li, G. H., Zhang, K. Q., Xu, J. P., Dong, J. Y., and Liu, Y. J. (2007). Nematicidal substances from fungi. Recent Pat. Biotechnol. 1, 212–233. doi: 10.2174/187220807782330165
Liu, X., Bimerew, M., Ma, Y., Muller, H., Ovadis, M., Eberl, L., et al. (2007). Quorum sensing signaling is required for production of the antibiotic pyrrolnitrin in a rhizospheric biocontrol strain of Serratia plymuthica. FEMS Microbiol. Lett. 270, 299–305. doi: 10.1111/j.1574-6968.2007.00681.x
Liu, Y., Chen, Z., Liu, L., Han, P., Wang, X., Li, S., et al. (2022). Broad-spectrum antifungal activity of lipopeptide brevilaterin B and its inhibition effects against Fusarium oxysporum and Penicillium chrysogenum. J. Appl. Microbiol. 132, 1330–1342. doi: 10.1111/jam.15285
Liu, K., McInroy, J. A., Hu, C. H., and Kloepper, J. W. (2018). Mixtures of plant-growth-promoting rhizobacteria enhance biological control of multiple plant diseases and plant-growth promotion in the presence of pathogens. Plant Dis. 102, 67–72. doi: 10.1094/pdis-04-17-0478-re
Llorente, B. E., Alasia, M. A., and Larraburu, E. E. (2016). Biofertilization with Azospirillum brasilense improves in vitro culture of Handroanthus ochraceus, a forestry, ornamental and medicinal plant. N. Biotechnol. 33, 32–40. doi: 10.1016/j.nbt.2015.07.006
Lobo, C. B., Juárez Tomás, M. S., Viruel, E., Ferrero, M. A., and Lucca, M. E. (2019). Development of low-cost formulations of plant growth-promoting bacteria to be used as inoculants in beneficial agricultural technologies. Microbiol. Res. 219, 12–25. doi: 10.1016/j.micres.2018.10.012
Lommen, S. T. E., Koops, K. G., Cornelder, B. A., de Jong, P. W., and Brakefield, P. M. (2019). Genetics and selective breeding of variation in wing truncation in a flightless aphid control agent. Entomol. Exp. Appl. 167, 636–645. doi: 10.1111/eea.12810
Lucas, J. (2011). Advance in plant disease and pest management. J. Agric. Sci. 149, 91–114. doi: 10.1017/S0021859610000997
Lugtenberg, B., and Kamilova, F. (2009). Plant growth promoting rhizobacteria. Annu. Rev. Microbiol. 63, 541–556. doi: 10.1146/annurev.micro.62.081307.162918
Lyu, D., Backer, R., Subramanian, S., and Smith, D. (2020). Phytomicrobiome coordination signals hold potential for climate change-resilient agriculture. Front. Plant Sci. 11:634. doi: 10.3389/fpls.2020.00634
Maksimov, I. V., Abizgil’dina, R. R., and Pusenkova, L. I. (2011). Plant growth promoting rhizobacteria as alternative to chemical crop protectors from pathogens (review). Appl. Biochem. Microbiol. 47, 333–345. doi: 10.1134/S0003683811040090
Maksimov, I. V., Blagova, D. K., Veselova, S. V., Sorokan, A. V., Burkhanova, G. F., and Cherepanova, E. A. (2020). Recombinant Bacillus subtilis 26DCryChS line with gene Btcry1Ia encoding Cry1Ia toxin from Bacillus thuringiensis promotes integrated wheat defense against pathogen Stagonospora nodorum Berk and greenbug Schizaphis graminum Rond. Biol. Control 144:104242. doi: 10.1016/j.biocontrol.2020.104242
Mathys, J., De Cremer, K., Timmermans, P., Van Kerckhove, S., Lievens, B., Vanhaecke, M., et al. (2012). Genome-wide characterization of ISR induced in Arabidopsis thaliana by Trichoderma hamatum T382 against Botrytis cinerea infection. Front. Plant Sci. 3:108. doi: 10.3389/fpls.2012.00108
Mau, Y. S., Prayetno, R. S., Kaka, H., Naat, K. D., Henuk, J. B. D., Hahuly, M. V., et al. (2022). Efficacy of indigenous Trichoderma isolates of West Timor, Indonesia, as biocontrol agents of brown spot (Drechslera oryzae) on two upland rice varieties. Egypt J. Biol. Pest Control 32, 1–10. doi: 10.1186/s41938-022-00559-x
Mauch-Mani, B., Baccelli, I., Luna, E., and Flors, V. (2017). Defense priming: an adaptive part of induced resistance. Annu. Rev. Plant Biol. 68, 485–512. doi: 10.1146/annurev-arplant-042916-041132
Maung, C. E. H., Baek, W. S., Choi, T. G., and Kim, K. Y. (2021). Control of grey mould disease on strawberry using the effective agent, Bacillus amyloliquefaciens Y1. Biocontrol Sci.Technol. 31, 468–482. doi: 10.1080/09583157.2020.1867707
McIntyre, J. L., and Press, L. S. (1991). “Formulation, delivery systems and marketing of biocontrol agents and plant growth promoting rhizobacteria (PGPR),” in The Rhizosphere and plant growth. Beltsville symposia in agricultural research. eds. D. L. Keister and P. B. Cregan (Dordrecht: Springer), 289–295.
Mengiste, T., Laluk, K., and AbuQamar, S. (2010). “Mechanisms of induced resistance against B. cinerea,” in Post-Harvest Pathology, Plant Pathology in the 21st Century. vol. 2 eds. D. Prusky and M. L. Gullino, (Dordrecht: Springer), 13–30.
Mishra, J., Tewari, S., Singh, S., and Arora, N. (2015). “Biopesticides: where we stand?” in Plant Microbes Symbiosis: Applied Facets. ed. N. Arora (New Delhi: Springer), 37–75. doi: 10.1007/978-81-322-2068-8_2
Mohammadi, P., Tozlu, E., Kotan, R., and Kotan, M. S. (2017). Potential of some bacteria for biological control of postharvest citrus green mold caused by Penicillum digitatum. Plant Prot. Sci. 53, 134–143. doi: 10.17221/55/2016-PPS
Mohan, L. K., Kurein, S., and Sreeja, P. (2017). Management of Meloidogyne incognita in Solenostemon rotundifolius (Poir) Morton. Indian J. Nematol. 47, 1–5.
Muller, H., and Berg, G. (2008). Impact of formulation procedures on the effect of the biocontrol agent Serratia plymuthica HROC48 on Verticillium wilt in oilseed rape. BioControl 53, 905–916. doi: 10.1007/s10526-007-9111-3
Müller, K., Kramer, S., Haslwimmer, H., Marhan, S., Scheunemann, N., Butenschön, O., et al. (2016). Carbon transfer from maize roots and litter into bacteria and fungi depends on soil depth and time. Soil Biol. Biochem. 93, 79–89. doi: 10.1016/j.soilbio.2015.10.015
Murugan, L., Krishnan, N., Venkataravanappa, V., Saha, S., Mishra, A. K., Sharma, B. K., et al. (2020). Molecular characterization and race identification of Fusarium oxysporum f. sp. lycopersici infecting tomato in India. 3 Biotech 10, 1–12. doi: 10.1007/s13205-020-02475-z
Musilova, L., Ridl, J., Polivkova, M., Macek, T., and Uhlik, O. (2016). Effects of secondary plant metabolites on microbial populations: changes in community structure and metabolic activity in contaminated environments. Int. J. Mol. Sci. 17:1205. doi: 10.3390/ijms17081205
Nabila, N., and Kasiamdari, R. S. (2021). Antagonistic activity of siderophore-producing bacteria from black rice rhizosphere against rice blast fungus Pyricularia oryzae. Microbiol. Biotechnol. Lett. 49, 217–224. doi: 10.48022/mbl.2011.11009
Nakashita, H., Yasuda, M., Nitta, T., Asami, T., Fujioka, S., Arai, Y., et al. (2003). Brassinosteroid functions in a broad range of disease resistance in tobacco and rice. Plant J. 33, 887–898. doi: 10.1046/j.1365-313x.2003.01675.x
Nally, M. C., Pesce, V. M., Maturano, Y. P., Muño, C. J., Combina, M., Toro, M. E., et al. (2012). Biocontrol of Botrytis cinerea in table grapes by non-pathogenic indigenous Saccharomyces cerevisiae yeasts isolated from viticultural environments in Argentina. Postharvest Biol. Technol. 64, 40–48. doi: 10.1016/j.postharvbio.2011.09.009
Nasima, I. A., Imran, A. S., Shahid, S. S., and Zaki, M. J. (2002). Nematicidal activity of some strains of Pseudomonas spp. Soil Biol. Biochem. 34, 1051–1058. doi: 10.1016/s0038-0717(02)00029-9
Nazari, M., and Smith, D. L. (2020). A PGPR-produced bacteriocin for sustainable agriculture: a review of thuricin 17 characteristics and applications. Front. Plant Sci. 11:916. doi: 10.3389/fpls.2020.00916
Nielsen, T. H., Sorensen, D., Tobiasen, C., Andersen, J. B., Christophersen, C., Givskov, M., et al. (2002). Antibiotic and biosurfactant properties of cyclic lipopeptides produced by fluorescent Pseudomonas spp. from the sugar beet rhizosphere. Appl. Environ. Microbiol. 68, 3416–3423. doi: 10.1128/AEM.68.7.3416-3423.2002
Nivedhitha, V. R., Shweta, B., Deepa, D. D., Manojkumar, N. H., and Raghavendra, R. B. (2008). Plant growth promoting microorganisms (PGPMs) from bamboo rhizosphere. J. Adv. Biotechnol. 7, 33–35.
Nunes, C. A. (2012). Biological control of postharvest diseases of fruit. Eur. J. Plant Pathol. 133, 181–196. doi: 10.1007/s10658-011-9919-7
O’Brien, P. A. (2017). Biological control of plant diseases. Australas. Plant Pathol. 46, 293–304. doi: 10.1007/s13313-017-0481-4
Ongena, M., and Jacques, P. (2008). Bacillus lipopeptides: versatile weapons for plant disease biocontrol. Trends Microbiol. 16, 115–125. doi: 10.1016/j.tim.2007.12.009
Pal, K. K., and Gardener, B. M. (2006). Biological control of plant pathogens. Plant Health Instr., 1–25. doi: 10.1094/PHI-A-2006-1117-02
Park, K., Park, J. W., Lee, S. W., and Balaraju, K. (2013). Induced suppression of soft rot disease in tobacco by combined application of Bacillus subtilis strain B4 and chemical elicitor BTH. Biocontrol Sci. Tech. 23, 968–979. doi: 10.1080/09583157.2013.811467
Parveen, G., Noreen, R., Shafique, H. A., Sultana, V., Ehteshamul-Haque, S., and Athar, M. (2019). Role of rhizobia in suppressing the root diseases of soybean under soil amendment. Planta Daninha 37:e019172336. doi: 10.1590/s0100-83582019370100038
Patkowska, E. (2021). Biostimulants managed fungal phytopathogens and enhanced activity of beneficial microorganisms in rhizosphere of Scorzonera (Scorzonera hispanica L.). Agriculture 11:347. doi: 10.3390/agriculture11040347
Pendse, M. A., Karwande, P. P., and Limaye, M. N. (2013). Past, present and future of nematophagous fungi as bio-agent to control plant parasitic nematodes. J. Plant Prot. Sci. 5, 1–9.
Peng, D., Li, S., Wang, J., Chen, C., and Zhou, M. (2014). Integrated biological and chemical control of rice sheath blight by Bacillus subtilis NJ-18 and jinggangmycin. Pest Manag. Sci. 70, 258–263. doi: 10.1002/ps.3551
Pérez-de-Luque, A., Tille, S., Johnson, I., Pascual-Pardo, D., Ton, J., and Cameron, D. D. (2017). The interactive effects of arbuscular mycorrhiza and plant growth-promoting rhizobacteria synergistically enhance host plant defences against pathogens. Sci. Rep. 7:16409. doi: 10.1038/s41598-017-16697-4
Pieterse, C. M., Zamioudis, C., Does, D. V., and Van Wees, S. (2014). “Signalling networks involved in induced resistance,” in Induced Resistance for Plant Defense: A Sustainable Approach to Crop Protection. eds. D. R. Walters, A. C. Newton, and G. D. Lyon (New York: John Wiley and Sons), 58–80.
Pii, Y., Mimmo, T., Tomasi, N., Terzano, R., Cesco, S., and Crecchio, C. (2015). Microbial interactions in the rhizosphere: beneficial influences of plant growth promoting rhizobacteria on nutrient acquisition process. A review. Biol. Fertil. Soils 51, 403–415. doi: 10.1007/s00374-015-0996-1
Prince, L., Raja, A., and Prabakaran, P. (2011). Antagonistic potentiality of some soil mycoflora against Colletotrichum falcatum. World J. Sci. Technol. 1, 39–42.
Priya, M. S., and Kumar, S. (2006). Dose optimization of Paecilomyces lilacinus for the control of Meloidogyne incognita on tomato. Indian J. Nematol. 36, 27–31.
Punja, Z. K., Rodriguez, G., and Tirajoh, A. (2016). Effects of Bacillus subtilis strain QST 713 and storage temperatures on post-harvest disease development on greenhouse tomatoes. Crop Prot. 84, 98–104. doi: 10.1016/j.cropro.2016.02.011
Punja, Z. K., and Utkhede, R. S. (2003). Using fungi and yeasts to manage vegetable crop diseases. Trends Biotechnol. 21, 400–407. doi: 10.1016/S0167-7799(03)00193-8
Qiao, J., Yu, X., Liang, X., Liu, Y., Borriss, R., and Liu, Y. (2017). Addition of plant-growth-promoting Bacillus subtilis PTS-394 on tomato rhizosphere has no durable impact on composition of root microbiome. BMC Microbiol. 17:131. doi: 10.1186/s12866-017-1039-x
Raaijmakers, J. M., and Mazzola, M. (2012). Diversity and natural functions of antibiotics produced by beneficial and plant pathogenic bacteria. Annu. Rev. Phytopathol. 50, 403–424. doi: 10.1146/annurev-phyto-081211-172908
Raaijmakers, J. M., Vlami, M., and de Souza, J. T. (2002). Antibiotic production by bacterial biocontrol agents. Antonie Van Leeuwenhoek 81, 537–547. doi: 10.1023/A:1020501420831
Rady, M. M., Boriek, S. H. K., Abd El-Mageed, T. A., Seif El-Yazal, M. A., Ali, E. F., Hassan, F. A. S., et al. (2021). Exogenous gibberellic acid or dilute bee honey boosts drought stress tolerance in Vicia faba by rebalancing osmoprotectants, antioxidants, nutrients, and phytohormones. Plants 10:748. doi: 10.3390/plants10040748
Radzki, W., Gutierrez Mañero, F., Algar, E., Lucas, J., García, J. A., García-Villaraco, A., et al. (2013). Bacterial siderophores efficiently provide iron to iron-starved tomato plants in hydroponics culture. Antonie Van Leeuwenhoek 104, 321–330. doi: 10.1007/s10482-013-9954-9
Rahman, A., Korejo, F., Sultana, V., Ara, J., and Ehteshamulhaque, S. (2017). Induction of systemic resistance in cotton by the plant growth promoting rhizobacterium and seaweed against charcoal rot disease. Pak. J. Bot. 49, 347–353.
Rao, M. S. (2007). Biopesticides for the management of nematodes in horticultural crops. Indian J. Plant Prot. 35, 202–205.
Raza, W., Ling, N., Yang, L., Huang, Q., and Shen, Q. (2016). Response of tomato wilt pathogen Ralstonia solanacearum to the volatile organic compounds produced by a biocontrol strain Bacillus amyloliquefaciens SQR-9. Sci. Rep. 6:24856. doi: 10.1038/srep24856
Rivera-Méndeza, W., Obregónc, M., Morán-Diezb, M. E., Hermosab, R., and Monteb, E. (2020). Trichoderma asperellum biocontrol activity and induction of systemic defenses against Sclerotium cepivorum in onion plants under tropical climate conditions. Biol. Control 141:104145. doi: 10.1016/j.biocontrol.2019.104145
Rosier, A., Medeiros, F. H. V., and Bais, H. P. (2018). Defining plant growth promoting rhizobacteria molecular and biochemical networks in beneficial plant-microbe interactions. Plant Soil 428, 35–55. doi: 10.1007/s11104-018-3679-5
Ruzzi, M., and Aroca, R. (2015). Plant growth-promoting rhizobacteria act as biostimulants in horticulture. Sci. Hortic. 196, 124–134. doi: 10.1016/j.scienta.2015.08.042
Saeed, E. E., Sham, A., Salmin, Z., Abdelmowla, Y., Iratni, R., El-Tarabily, K., et al. (2017). Streptomyces globosus UAE1, a potential effective biocontrol agent for black scorch disease in date palm plantations. Front. Microbiol. 8:1455. doi: 10.3389/fmicb.2017.01455
Salomon, M. V., Funes Pinter, I., Piccoli, P., and Bottini, R. (2017). “Use of plant growth-promoting rhizobacteria as biocontrol agents: induced systemic resistance against biotic stress in plants,” in Microbial applications: Biomedicine, agriculture and industry. ed. V. C. Kalia (Cham: Springer International Publishing), 133–152. doi: 10.1007/978-3-319-52669-0_7
Sánchez-Montesinos, B., Mila Santos, M., Moreno-Gavíra, A., Marín-Rodulfo, T., Gea, F. J., and Diánez, F. (2021). Biological control of fungal diseases by Trichoderma aggressivum f. europaeum and its compatibility with fungicides. J. Fungi 7:598. doi: 10.3390/jof7080598
Sanzani, S., Reverberi, M., and Geisen, R. (2016). Mycotoxins in harvested fruits and vegetables: insights in producing fungi, biological role, conducive conditions, and tools to manage postharvest contamination. Postharvest Biol. Technol. 122, 95–105. doi: 10.1016/j.postharvbio.2016.07.003
Savary, S., Willocquet, L., Pethybridge, S. J., Esker, P., McRoberts, N., and Nelson, A. (2019). The global burden of pathogens and pests on major food crops. Nat. Ecol. Evol. 3, 430–439. doi: 10.1038/s41559-018-0793-y
Savchuk, S., and Fernando, W. D. D. (2004). Effect of timing of application and population dynamics on the degree of biological control of Sclerotinia sclerotiorum by bacterial antagonists. FEMS Microbiol. Ecol. 49, 379–388. doi: 10.1016/j.femsec.2004.04.014
Sham, A., Al-Ashram, H., Whitley, K., Iratni, R., El-Tarabily, K. A., and AbuQamar, S. F. (2019). Metatranscriptomic analysis of multiple environmental stresses identifies RAP2.4 gene associated with Arabidopsis immunity to Botrytis cinerea. Sci. Rep. 9:17010. doi: 10.1038/s41598-019-53694-1
Sham, A., Moustafa, K., Al-Shamisi, S., Alyan, S., Iratni, R., and AbuQamar, S. F. (2017). Microarray analysis of Arabidopsis WRKY33 mutants in response to the necrotrophic fungus Botrytis cinerea. PLoS One 12:e0172343. doi: 10.1371/journal.pone.0172343
Shanthiyaa, V., Karthikeyan, G., and Raguchander, T. (2013). Production of extracellular proteins, cellulases and antifungal metabolites by Chaetomium globosum Kunze ex. Fr. Arch. Phytopathol. Plant Prot. 47, 517–528. doi: 10.1080/03235408.2013.813124
Sharf, R., Shiekh, H., Syed, A., Akhtar, A., and Robab, A. (2014). Interaction between Meloidogyne incognita and Pochonia chlamydosporia and their effects on the growth of Phaseolus vulgaris. Arch. Phytopathol. Plant Prot. 47, 622–630. doi: 10.1080/03235408.2013.816459
Shen, X., Hu, H., Peng, H., Wang, W., and Zhang, X. (2013). Comparative genomic analysis of four representative plant growth-promoting rhizobacteria in Pseudomonas. BMC Genomics 14:271. doi: 10.1186/1471-2164-14-271
Shivlata, L., and Satyanarayana, T. (2017). “Actinobacteria in agricultural and environmental sustainability,” in Agro-Eenvironmental Sustainability. eds. J. S. Singh and G. Seneviratne (Berlin: Springer), 173–218.
Siddiqui, I. A., and Shaukat, S. S. (2004). Trichoderma harzianum enhances the production of nematicidal compounds in vitro and improves biocontrol of Meloidogyne javanica by Pseudomonas fluorescens in tomato. Lett. Appl. Microbiol. 38, 169–175. doi: 10.1111/j.1472-765x.2003.01481.x
Silva, S. D., Carneiro, R. M. D. G., Faria, M., Souza, D. A., Monnerat, R. G., and Lopes, R. B. (2017). Evaluation of Pochonia chlamydosporia and Purpureocillium lilacinum for suppression of Meloidogyne enterolobii on tomato and banana. J. Nematol. 49, 77–85. doi: 10.21307/jofnem-2017-047
Singh, S., Prem, D., and Tanwar, R. S. (2005). Production and antifungal activity of secondary metabolites of Trichoderma virens. Pestic. Res. J. 17, 26–29.
Singh, N., Singh, D., and Singh, N. (2017). Effect of Glomus bagyaraji inoculation and phosphorus amendments on Fusarium wilt of chickpea. Agric. Res. J. 54, 236–243. doi: 10.5958/2395-146X.2017.00043.6
Sivasakthi, S., Usharani, G., and Saranraj, P. (2014). Biocontrol potentiality of plant growth promoting bacteria (PGPR)-Pseudomonas fluorescens and Bacillus subtilis. A review. Afr. J. Agric. Res. 9, 1265–1277. doi: 10.5897/AJAR
Snehalatharani, A., Devappa, V., and Sangeetha, C. G. (2021). “Postharvest diseases of banana and their management,” in Postharvest Handling and Diseases of Horticultural Produce. eds. D. Singh, R. R. Sharma, V. Devappa, and D. Kamil (Boca Raton: CRC Press), 201–210.
Spadaro, D., and Gullino, M. L. (2005). Improving the efficacy of biocontrol agents against soil borne pathogens. Crop Prot. 24, 601–613. doi: 10.1016/j.cropro.2004.11.003
Spaepen, S., Bossuyt, S., Engelen, K., Marchal, K., and Vanderleyden, J. (2014). Phenotypical and molecular responses of Arabidopsis thaliana roots as a result of inoculation with the auxin-producing bacterium Azospirillum brasilense. New Phytol. 201, 850–861. doi: 10.1111/nph.12590
Stirling, G. R. (2017). “Biological control of plant-parasitic nematodes,” in Diseases of Nematodes. eds. G. O. Poinar and H. B. Jansson (Boca Raton: CRC Press), 103–150.
Subramanian, S., and Smith, D. L. (2015). Bacteriocins from the rhizosphere microbiome: from an agriculture perspective. Front. Plant Sci. 6:909. doi: 10.3389/fpls.2015.00909
Sui, Y., Wang, Z., Zhang, D., and Wang, Q. (2021). Oxidative stress adaptation of the antagonistic yeast, Debaryomyces hansenii, increases fitness in the microenvironment of kiwi fruit wound and biocontrol efficacy against postharvest diseases. Biol. Control 152:104428. doi: 10.1016/j.biocontrol.2020.104428
Sultana, F., and Hossain, M. M. (2022). Assessing the potentials of bacterial antagonists for plant growth promotion, nutrient acquisition, and biological control of southern blight disease in tomato. PLoS One 17:e0267253. doi: 10.1371/journal.pone.0267253
Takishita, Y., Charron, J. B., and Smith, D. L. (2018). Biocontrol rhizobacterium Pseudomona sp. 23s induces systemic resistance in tomato (Solanum lycopersicum L.) against bacterial canker Clavibacter michiganensis subsp. michiganensis. Front. Microbiol. 9:2119. doi: 10.3389/fmicb.2018.02119
Tariq, M., Khan, A., Asif, M., Khan, F., Ansari, T., Shariq, M., et al. (2020). Biological control: a sustainable and practical approach for plant disease management. Acta Agric. Scand. B Soil Plant Sci. 70, 507–524. doi: 10.1080/09064710.2020.1784262
Tariq, M., Khan, A., Asif, M., and Siddiqui, M. A. (2018). Interactive effect of Trichoderma virens and Meloidogyne incognita and their influence on plant growth character and nematode multiplication on Abelmoschus esculentus (L.) Moench. Current Nematol. 29, 1–9.
Timper, P. (2011). “Utilization of biological control for managing plant-parasitic nematodes,” in Biological Control of Plant-Parasitic Nematodes: Building Coherence Between Microbial Ecology and Molecular Mechanisms. eds. K. Davies and S. Spiegel (Dordrecht: Springer), 259–289.
Torres, M. J., Brandan, C. P., Petroselli, G., Erra-Balsells, R., and Audisio, M. C. (2016). Antagonistic effects of Bacillus subtilis subsp., subtilis and B. amyloliquefaciens against Macrophomina phaseolina: SEM study of fungal changes and UV-MALDI-TOF MS analysis of their bioactive compounds. Microbiol. Res. 182, 31–39. doi: 10.1016/j.micres.2015.09.005
Tsegaye, Z., Assefa, F., Tefera, G., Alemu, T., Gizaw, B., and Abatenh, E. (2018). Concept, principle and application of biological control and their role in sustainable plant diseases management strategies. Int. J. Res. Stud. Biosci. 6, 18–34. doi: 10.20431/2349-0365.0604004
Usman, A., and Siddiqui, M. A. (2012). Effect of some fungal strains for the management of root-knot nematode (Meloidogyne incognita) on eggplant (Solanum melongena). J. Agric. Technol. 8, 213–218.
Vacheron, J., Desbrosses, G., Bouffaud, M. L., Touraine, B., MoenneLoccoz, Y., Muller, D., et al. (2013). Plant growth-promoting rhizobacteria and root system functioning. Front. Plant Sci. 4:356. doi: 10.3389/fpls.2013.00356
Vejan, P., Abdullah, R., Khadiran, T., Ismail, S., and Nasrulhaq Boyce, A. (2016). Role of plant growth promoting rhizobacteria in agricultural sustainability: a review. Molecules 21:573. doi: 10.3390/molecules21050573
Viaene, N., Coyne, D. L., and Kerry, B. R. (2006). “Biological and cultural management,” in Plant nematology. eds. R. N. Perry and M. Moens (Wallingford: CABI), 346–369.
Viejobueno, J., Albornoz, P. L., Camacho, M., de los Santos, B., Martínez-Zamora, M. G., and Salazar, S. M. (2021). Protection of strawberry plants against charcoal rot disease (Macrophomina phaseolina) induced by Azospirillum brasilense. Agronomy 11:195. doi: 10.3390/agronomy11020195
Vinale, F., Flematti, G., Sivasithamparam, K., Lorito, M., Marra, R., Skelton, B. W., et al. (2009). Harzianic acid, an antifungal and plant growth promoting metabolite from Trichoderma harzianum. J. Nat. Prod. 72, 2032–2035. doi: 10.1021/np900548p
Vitorino, L. C., Silva, F., Cruvinel, B. G., Bessa, L. A., Rosa, M., Souchie, E. L., et al. (2020). Biocontrol potential of Sclerotinia sclerotiorum and physiological changes in soybean in response to Butia archeri palm rhizobacteria. Plan. Theory 9:64. doi: 10.3390/plants9010064
Vos, C. M., Tesfahun, A. N., Panis, B., De Waele, D., and Elsen, A. (2012). Arbuscular mycorrhizal fungi induce systemic resistance in tomato against the sedentary nematode Meloidogyne incognita and the migratory nematode Pratylenchus penetrans. Appl. Soil Ecol. 61, 1–6. doi: 10.1016/j.apsoil.2012.04.007
Wallace, R. L., Hirkala, D. L., and Nelson, L. M. (2018). Efficacy of Pseudomonas fluorescens for control of Mucor rot of apple during commercial storage and potential modes of action. Can. J. Microbiol. 64, 420–431. doi: 10.1139/cjm-2017-0776
Wang, M., Geng, L., Sun, X., Shu, C., Song, F., and Zhang, J. (2020). Screening of Bacillus thuringiensis strains to identify new potential biocontrol agents against Sclerotinia sclerotiorum and Plutella xylostella in Brassica campestris L. Biol. Control 145, 104262–104269. doi: 10.1016/j.biocontrol.2020.104262
Watanabe, A., Kamei, K., Sekine, T., Waku, M., Nishimura, K., Miyaji, M., et al. (2004). Effect of aeration on gliotoxin production by Aspergillus fumigatus in its culture filtrate. Mycopathologia 157, 19–27. doi: 10.1023/b:myco.0000012224.49131.dd
Weller, D. M. (2007). Pseudomonas biocontrol agents of soil borne pathogens: looking back over 30 years. Phytopathology 97, 250–256. doi: 10.1094/PHYTO-97-2-0250
Whipps, J. M. (2001). Microbial interactions and biocontrol in the rhizosphere. J. Exp. Bot. 52, 487–511. doi: 10.1093/jexbot/52.suppl_1.487
Yao, X., Huang, K., Zhao, S., Cheng, Q., Zhang, S., Yang, L., et al. (2021). Identification and verification of rhizosphere indicator microorganisms in tobacco root rot. Agron. J. 113, 1480–1491. doi: 10.1002/agj2.20547
Yones, A. M., and kayim, M. (2021). Molecular characterization of Trichoderma spp. with biocontrol ability against faba bean chocolate spot (Botrytis cinerea Pers. ex Fr.). Plant Cell Biotechnol. Mol. Biol. 22, 52–63.
Zamioudis, C., and Pieterse, C. M. (2012). Modulation of host immunity by beneficial microbes. Mol. Plant Microbe Interact. 25, 139–150. doi: 10.1094/MPMI-06-11-0179
Zapata-Sarmiento, D. H., Palacios-Pala, E. F., Rodríguez-Hernández, A. A., Melchor, D. L. M., Rodríguez-Monroy, M., and Sepúlveda-Jiménez, G. (2020). Trichoderma asperellum, a potential biological control agent of Stemphylium vesicarium, on onion (Allium cepa L.). Biol. Control 140:104105. doi: 10.1016/j.biocontrol.2019.104105
Zgadzaj, R., Garrido-Oter, R., Jensen, D. B., Koprivova, A., SchulzeLefert, P., and Radutoiu, S. (2016). Root nodule symbiosis in Lotus japonicus drives the establishment of distinctive rhizosphere, root, and nodule bacterial communities. Proc. Natl. Acad. Sci. USA 113, E7996–E8005. doi: 10.1073/pnas.1616564113
Zhang, Y., Li, T., Xu, M., Guo, J., Zhang, C., Feng, Z., et al. (2021b). Antifungal effect of volatile organic compounds produced by Pseudomonas chlororaphis subsp. aureofaciens SPS-41 on oxidative stress and mitochondrial dysfunction of Ceratocystis fimbriata. Pestic. Biochem. Physiol. 173:104777. doi: 10.1016/j.pestbp.2021.104777
Zhang, H., Liu, Y., and Wang, G. (2019). Integrated use of maize bran residue for one-step phosphate bio-fertilizer production. Appl. Biochem. Biotechnol. 187, 1475–1487. doi: 10.1007/s12010-018-2874-4
Zhang, Q., Stummer, B. E., Guo, Q., Zhang, W., Zhang, X., Zhang, L., et al. (2021a). Quantification of Pseudomonas protegens FD6 and Bacillus subtilis NCD-2 in soil and the wheat rhizosphere and suppression of root pathogenic Rhizoctonia solani AG-8. Biol. Control 154:104504. doi: 10.1016/j.biocontrol.2020.104504
Zhang, S., and Zhang, X. (2009). Effects of two composted plant pesticide residues, incorporated with Trichoderma viride on root-knot nematode in Balloonflower. Agric. Sci. China 8, 447–454. doi: 10.1016/S1671-2927(08)60231-X
Keywords: biofertiIizers, biopestcide, crop yield, disease suppression, pathogen suppression, plant growth-promoting rhizhobacteria
Citation: El-Saadony MT, Saad AM, Soliman SM, Salem HM, Ahmed AI, Mahmood M, El-Tahan AM, Ebrahim AAM, Abd El-Mageed TA, Negm SH, Selim S, Babalghith AO, Elrys AS, El-Tarabily KA and AbuQamar SF (2022) Plant growth-promoting microorganisms as biocontrol agents of plant diseases: Mechanisms, challenges and future perspectives. Front. Plant Sci. 13:923880. doi: 10.3389/fpls.2022.923880
Edited by:
Nikolay Vassilev, University of Granada, SpainReviewed by:
Joginder Singh, Lovely Professional University, IndiaStefany Castaldi, University of Naples Federico II, Italy
Copyright © 2022 El-Saadony, Saad, Soliman, Salem, Ahmed, Mahmood, El-Tahan, Ebrahim, Abd El-Mageed, Negm, Selim, Babalghith, Elrys, El-Tarabily and AbuQamar. This is an open-access article distributed under the terms of the Creative Commons Attribution License (CC BY). The use, distribution or reproduction in other forums is permitted, provided the original author(s) and the copyright owner(s) are credited and that the original publication in this journal is cited, in accordance with accepted academic practice. No use, distribution or reproduction is permitted which does not comply with these terms.
*Correspondence: Khaled A. El-Tarabily, ktarabily@uaeu.ac.ae; Synan F. AbuQamar, sabuqamar@uaeu.ac.ae